Understanding Energy Quanta: Insights and Applications
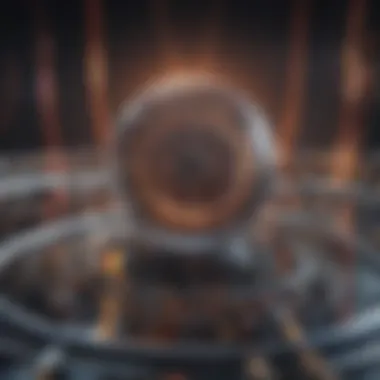
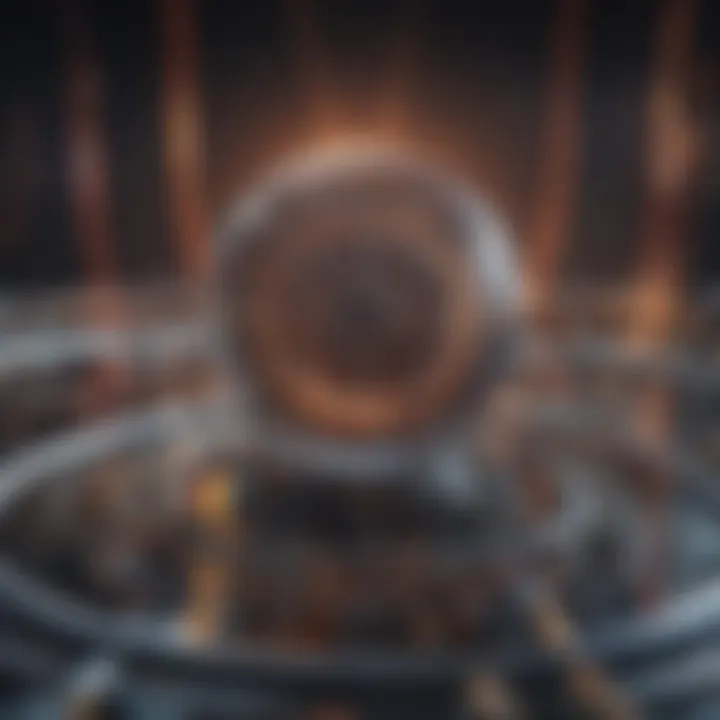
Intro
The exploration of energy at the quantum level is both complex and fascinating. The concept of energy quantization fundamentally shifts our understanding from classical mechanics, where energy is thought to be continuous. In quantum mechanics, energy exists in discrete units called quanta. This opens a new frontier in both theoretical study and practical application.
In this article, we unpack the concept of quanta of energy. By examining historical developments and key principles in the field, we highlight significant advancements in research and their implications. Understanding the nuances of quanta fosters a deeper comprehension of phenomena spanning from atomic interactions to the evolution of modern technology.
The implications of energy quantization extend far beyond theoretical discussions. They influence innovative applications in various scientific fields such as quantum computing, telecommunications, and material sciences. Welcome to an insightful discussion that strives to bridge the gap between abstract concepts and their tangible consequences.
Key Research Findings
Overview of Recent Discoveries
Recent studies have provided exciting insights into the realm of energy quantization. The discoveries around blackbody radiation and the photoelectric effect have clarified how energy quantizes at the atomic level. The work of Max Planck and Albert Einstein laid vital groundwork, showing that light can behave both as a particle and a wave.
More recent advancements, especially in quantum field theory, further reveal how particles interact and exchange energy in quantized forms. These breakthroughs are significant as they not only enhance foundational knowledge but also pave the way for technology that utilizes these principles.
Significance of Findings in the Field
The implications of these findings go beyond academia. Technologies like lasers and semiconductors rely on the principles of energy quantization. Additionally, understanding quanta is essential for developments in sensor technologies and the ongoing progress in quantum computing, which promises unparalleled processing capabilities.
"At the heart of quantum theory lies the concept of quantization, fundamentally transforming our approach to technology and understanding nature."
Moreover, energy quantization impacts our comprehension of the universe. Insights gleaned from quantum mechanics enhance our understanding of cosmological phenomena, such as black holes and the origin of cosmic background radiation. We gain a clearer view of how the universe operates at its most fundamental level.
Breakdown of Complex Concepts
Simplification of Advanced Theories
Energy quantization can often feel abstract. However, breaking it down into simpler theories makes it more comprehensible. For instance, the fundamental idea that energy levels in an atom are discrete can be demonstrated through models and equations that illustrate electron transitions between levels.
Visual Aids and Infographics
Visual representations prove beneficial in explaining complex theories. Infographics illustrating the quantization of energy levels provide clarity. Graphs showing emission or absorption spectra of elements can visually depict how energy quanta correspond to specific transitions in atoms.
By utilizing these methods, we can effectively convey intricate concepts to readers, enabling a broader understanding of the significance of energy quantization.
Foundations of Energy Quantization
The quest for understanding energy at its most fundamental level begins with the concept of quantization. This underpins various fields in physics, chemistry, and technology. It helps explain phenomena that traditional approaches fail to elucidate. Knowing about energy quantization is crucial for both theoretical pursuits and practical applications.
Historical Context
The notion of quantization arose in the early 20th century, challenging classical physics. Max Planck, in 1900, introduced the idea during his work on blackbody radiation. In an attempt to resolve inconsistencies in heat distribution, he proposed that energy is emitted or absorbed in discrete units or 'quanta'. This breakthrough laid the groundwork for quantum theory.
Leading into the 1920s, further developments by scientists like Niels Bohr and Albert Einstein expanded on these ideas. They explored atomic structures and photon interactions, highlighting how quantization is not a mere abstract concept but a fundamental aspect of nature itself. Effective understanding of historical context enables students and scholars to appreciate how our comprehension of energy has evolved. The mistakes and corrections of the past guide present and future research.
Key Definitions and Concepts
Quanta in Physics
Quanta in physics defines the smallest possible discrete unit of any physical property. For example, light can be described in terms of photons, each representing a quantized amount of energy. This concept is pivotal because it addresses phenomena, such as the photoelectric effect, which cannot be explained using classical physics alone.
A key characteristic of quanta is their intrinsic ability to define states in quantum mechanics. This distinction enables physicists to predict behaviors in systems where probabilities reign. Such insights make exploring non-classical states advantageous in areas like quantum computing.
Nonetheless, the challenge lies in the non-intuitive nature of quanta. Their behavior often strays from human experience, leading to debates on observability and measurement. Nonetheless, quanta remain vital for integrating theory with practical applications.
Energy Levels
Energy levels refer to the specific quantized states that particles, particularly electrons in atoms, can occupy. Each level has a distinct energy value. The significance of energy levels is paramount, as they explain atomic stability and behavior during energy transactions.
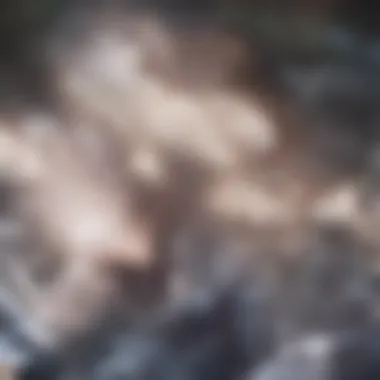
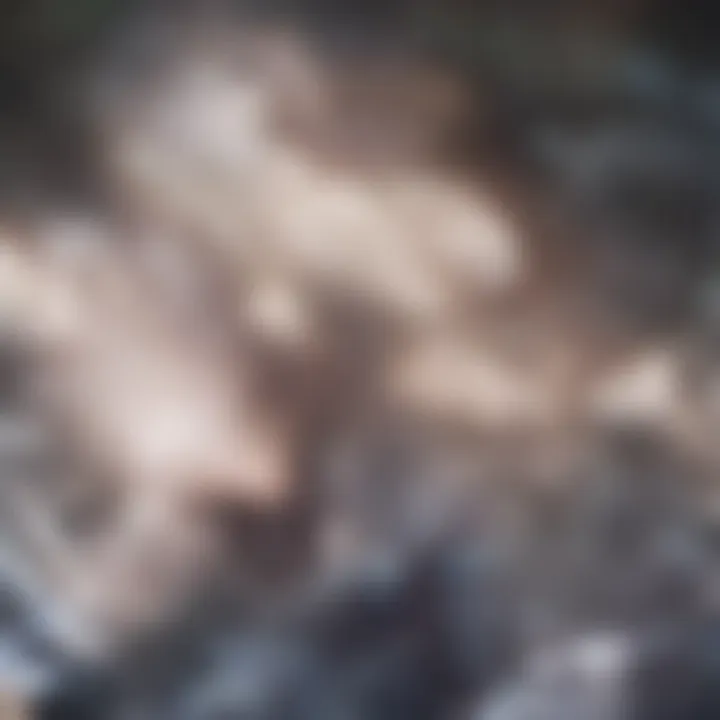
The ability to understand energy levels enriches discussions concerning chemical bonds, spectral emissions, and quantum transitions. For instance, knowing the energy levels of electrons helps in designing semiconductors, critical for modern electronics.
However, the complexity increases with multi-electron systems. As electrons interact, energy level determination becomes intricate. Yet, the utility of understanding these levels in practical applications justifies the effort.
Planck's Hypothesis
Planck's hypothesis revolutionized thermal physics. By asserting that energy is quantized, it introduced a new framework necessary for tackling issues at atomic and subatomic levels. This principle implies that physical systems do not absorb or emit energy continuously but rather in fixed amounts, influencing subsequent theories.
Planck's constant is a fundamental aspect derived from his hypothesis, which has since become a critical constant in quantum mechanics. It signifies the small-scale measurements crucial for understanding microscopic behaviors.
In summary, the foundations of energy quantization not only present the phenomena observed but also guide the technological implementations arising from quantum insights. This section reveals how energy structures not just shape scientific discourse but also enhance our interfacing with nature.
The Role of Quanta in Quantum Mechanics
The discussion of energy quanta is central to the understanding of quantum mechanics. It redefines classical concepts of energy and matter. Unlike classical physics, where energy can take any value, the quantum theory posits that energy exists in discrete units, known as quanta. This change in perspective significantly shifts how scientists perceive interactions at the atomic and subatomic levels.
It is important to recognize the implications of these concepts. By examining the properties of quanta, researchers can delve into how energy operates in a way that differs fundamentally from classical predictions. This understanding not only helps in deciphering complex phenomena but also aids in the development of technologies based on quantum principles.
Wave-Particle Duality
Wave-particle duality is a fundamental concept in quantum mechanics that illustrates how particles, such as electrons and photons, can exhibit both wave-like and particle-like properties. This duality challenges conventional views by merging two seemingly opposing behaviors. When conducting experiments, particles can behave like waves, creating interference patterns. However, under different conditions, they also demonstrate properties typical of particles, such as localized collisions.
This dual nature has far-reaching consequences in interpreting quantum phenomena. For instance, it raises questions about the very nature of reality and observation, influencing how measurements are perceived in quantum mechanics. Understanding wave-particle duality is crucial for grasping more complex topics, including the behavior of matter and energy at tiny scales.
Energy Measurement in Quantum Systems
Energy measurement in quantum systems is inherently linked to the uncertainty principle, which states that certain pairs of physical properties cannot be simultaneously known to arbitrary precision. As a result, when measuring a particle's energy, one inevitably disturbs its momentum. This introduces fundamental limits to the accuracy of measurements, a departure from the deterministic nature of classical physics.
In quantum mechanics, energy is quantized, meaning that systems can only exist in specific energy states. For example, electrons in an atom occupy distinct energy levels. Thus, measuring these energy levels can reveal much about the system itself, including the possible transitions between states and the absorption or emission of energy.
Quantum States and Superposition
Quantum states describe the various configurations of a quantum system. According to quantum mechanics, particles can exist in multiple states at once, a phenomenon termed superposition. This can be illustrated through the famous thought experiment involving Schrรถdinger's cat, where the cat can be simultaneously both alive and dead until observed.
Superposition is foundational to understanding quantum computation and other advanced applications. In simple terms, while a classic bit can be either 0 or 1, a qubit can represent 0, 1, or both at the same time, vastly increasing computational power.
The implications of superposition lead researchers to consider how to manage complex quantum systems and utilize them effectively in technology, further emphasizing the importance of energy quanta in the broader context of quantum mechanics.
The understanding of quanta in quantum mechanics not only reshapes our view of the universe but also drives advancements in various technological fields.
Implications of Energy Quantization
The study of energy quantization has substantial implications across various fields of physics and technology. Energy quantization refers to the idea that energy can only exist in discrete amounts, or quanta, rather than any arbitrary value. This concept is central to how we understand atomic and subatomic systems. It provides a framework that helps to bridge theoretical concepts with practical applications.
One critical implication is its foundational role in understanding the behavior of matter at microscopic scales. It allows scientists to extrapolate behavior in quantum systems, impacting everything from theoretical physics to practical engineering designs.
Furthermore, energy quantization directly influences advancements in technology. Quantum mechanics leads to innovative computing solutions and new methods for harnessing energy. Therefore, recognizing these implications is significant for scientific progress and our comprehension of the physical universe.
Fundamental Physics
The Uncertainty Principle
The Uncertainty Principle, introduced by Werner Heisenberg, is a crucial aspect of quantum mechanics. It states that certain pairs of physical properties cannot be measured precisely at the same time. For example, the position and momentum of a particle cannot both be known exactly. This principle illuminates the limits of measurement and knowledge in quantum systems.
Its key characteristic is that it challenges classical intuitions about precision in measurement. This makes it a popular choice for discussions in this article. It underscores the inherent limitations within quantum physics, which can be both an advantage and a disadvantage in real-world applications. While it provides insight into the behavior of particles, it can also complicate the design of experiments and technologies.
Energy Conservation Laws
Energy Conservation Laws dictate that the total energy in a closed system remains constant. This principle remains fundamental in both classical and modern physics. It signifies that energy cannot be created or destroyed, only transformed from one form to another. This characteristic makes the law a cornerstone of physics and a beneficial topic for discussion.
A unique feature of these laws is their role in both macroscopic and quantum systems. They link various phenomena, establishing a continuity in our understanding of energy transitions. This consistency is advantageous when theorizing about complex interactions in quantum mechanics and thermodynamics.
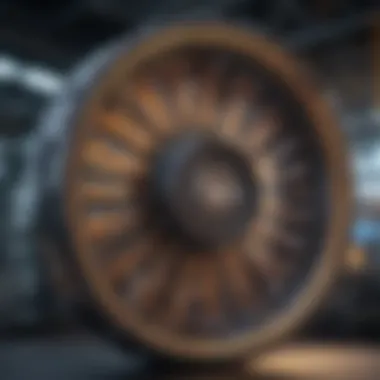
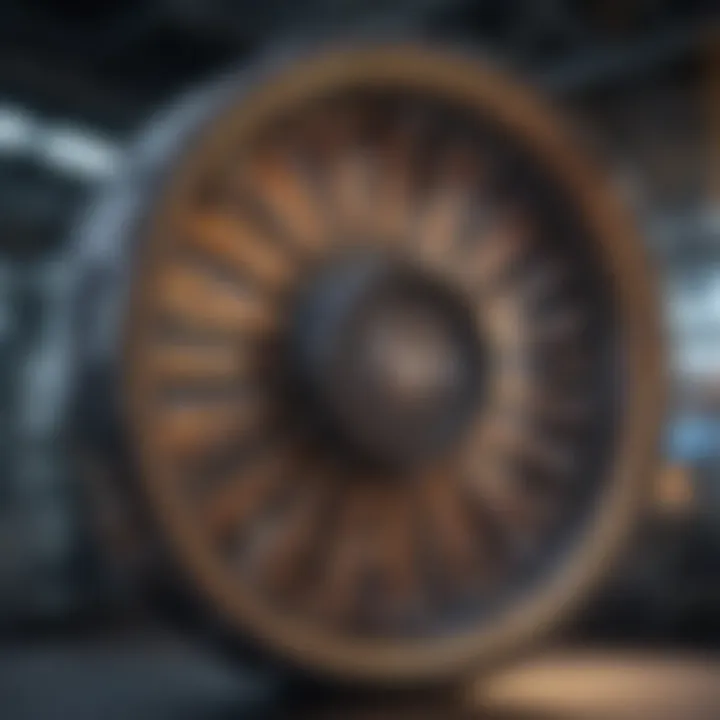
Technological Advancements
Quantum Computing
Quantum Computing represents a significant advancement made possible by the principles of energy quantization. It utilizes quantum bits, or qubits, which can exist in multiple states simultaneously, thus enabling computation at velocities unattainable by classical computers. This method can enhance performance in complex calculations.
One key characteristic is the potential for exponential speedup in solving certain types of problems. This makes Quantum Computing a compelling focus of this article. The unique feature of quantum superposition allows for parallel processing, making it a powerful tool in data science, cryptography, and more. Despite its promises, challenges such as error rates and the requirement for stable quantum states pose disadvantages to widespread application.
Photovoltaics
Photovoltaics involve the conversion of light into electricity using semiconducting materials that exhibit energy quantization. This technology is not only crucial for sustainable energy but also illustrates the practical applications of quantum mechanics. It takes advantage of the photoelectric effect, where photons dislodge electrons, resulting in electrical current.
The essential feature of photovoltaics lies in their ability to convert sunlight into usable energy efficiently. This characteristic has made them popular and practical in various industries. However, challenges remain, including energy efficiency and the need for storage solutions for intermittent energy sources.
The Relationship with Thermodynamics
The interplay between quantum mechanics and thermodynamics offers profound insights into how energy operates at both large and small scales. Understanding quantization in energy leads to refined interpretations of thermodynamic laws, especially when considering systems at thermal equilibrium versus non-equilibrium states.
Key points of this relationship include:
- Thermal Energy Distribution: Energy quantization alters the ways in which thermal energy is distributed among particles in a system.
- Statistical Mechanics: Quantum mechanics informs statistical mechanics, which connects microscopic particle behavior with macroscopic thermodynamic properties.
- Entropy: The concept of entropy is influenced by quantum states and energy levels, offering fresh perspectives on disorder and organization in systems.
In summary, the implications of energy quantization extend far beyond theoretical discussions. They directly affect our understanding of foundational physics, prompt technological advancements, and guide our perception of thermodynamic relationships.
Quanta of Energy in Nature
Understanding the quanta of energy in nature is crucial for grasping how energy interacts within various systems. This section emphasizes how fundamental concepts of energy quantization apply broadly across disciplines such as chemistry and biology. By focusing on specific phenomena, we can appreciate the intricate roles that energy play in forming the foundation of natural processes.
Quantum Phenomena in Chemistry
Quantum mechanics significantly informs our understanding of chemical processes. The energy at the quantum level dictates how atoms and molecules behave, particularly through two primary aspects: molecular orbitals and chemical reactions.
Molecular Orbitals
Molecular orbitals are essential for describing the distribution of electrons in molecules. They arise from the combination of atomic orbitals when atoms bond together. One key characteristic of molecular orbitals is their ability to indicate the shapes and energies associated with electrons, providing insights into chemical bonding.
The utility of molecular orbitals lies in their detailed representation of electron interactions, making them a popular model for predicting molecular behavior. This feature allows scientists to accurately estimate the energy levels involved in the formation of bonds, thereby influencing reactivity and stability.
However, one must also consider that molecular orbitals can introduce complexity in calculations and modeling. These intricacies may require advanced computational resources and specialized knowledge to analyze correctly. In this article, we explore their contributions to energy quantization and the implications of this understanding in more significant chemical contexts.
Chemical Reactions
Chemical reactions are another vital component that derives its essence from the quanta of energy. In essence, these reactions involve the breaking and forming of bonds, where energy changes take place at the quantum level. The key characteristic here is how energy is absorbed or released, affecting the reactionโs dynamics.
The significance of chemical reactions in this discussion cannot be overstated. They are central to industrial processes, biological interactions, and environmental chemistry. Understanding energy quantization allows chemists to manipulate reactions more effectively, leading to improved efficiencies and innovative products. Yet, the unique features of these processes, such as activation energy thresholds, often complicate the understanding of reaction mechanics. Consequently, this can pose challenges in predicting outcomes without rigorous experimentation.
Biological Systems and Energy Transfer
Energy transfer within biological systems also heavily reflects the principles of quantized energy. This section will discuss photosynthesis and cellular respiration as two fundamental biological processes underpinned by the quantum nature of energy.
Photosynthesis
Photosynthesis is a process where plants convert light energy into chemical energy. The specific aspect of energy quantization is pivotal here, as it allows plants to absorb photons and utilize this energy in bonding carbon dioxide and water to create glucose. This process operates under strictly defined energy levels, emphasizing the significance of quanta.
A crucial characteristic of photosynthesis is its efficiency in harnessing solar energy. This allows life on Earth to exist and thrive. By focusing on light at particular wavelengths, plants are adept at capturing energy, a unique feature that highlights the elegance of energy quantization in nature.
However, the dependency on specific wavelengths can sometimes limit photosynthetic efficiency under varying light conditions, demonstrating the need for further understanding of these mechanisms. Nevertheless, exploring these nuances enriches our knowledge of energy dynamics within living organisms.
Cellular Respiration
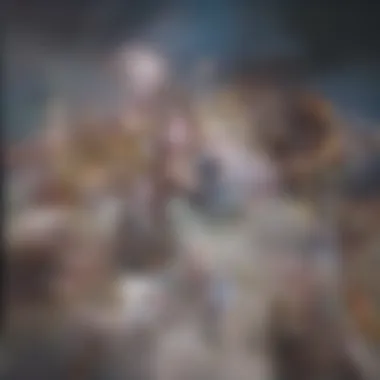
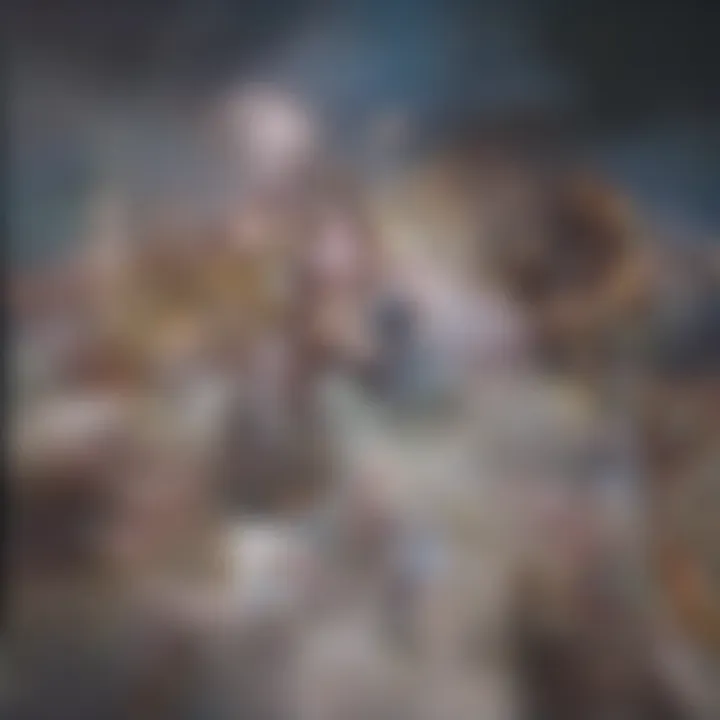
Cellular respiration is the process by which cells convert biochemical energy from nutrients into adenosine triphosphate (ATP), utilizing oxygen in the process. The quantized nature of energy plays a significant role, as specific energy changes occur during glucose breakdown to release ATP.
One key characteristic of cellular respiration is its universality across all forms of life. This feature showcases how energy quantization governs essential life processes. ATP, being the universal energy currency, highlights the implications of energy quantization in both efficiency and regulation of metabolic pathways.
Nonetheless, the complexity of cellular respiration can pose challenges in understanding the efficiency of energy transfer within cells. The intricate layers of feedback mechanisms and metabolic regulation necessitate extensive research for optimization. This further illustrates the profound relationship between energy quantization and biological systems, stressing continuous exploration of these critical processes.
Challenges and Future Directions
The exploration of energy quantization presents many challenges. Understanding these limitations helps identify paths for future inquiry. This article does not merely present established facts but probes into the complexities that lie ahead in this scientific domain.
Limitations of Current Understanding
Despite considerable achievements in quantum mechanics, researchers face significant gaps. Our understanding of energy quantization is often theoretical. Many principles do not yet have experimental verification, which can create uncertainty in practical applications. For example, while quantum entanglement is well-documented, how it affects energy transfer in practical systems remains unclear.
Also, advancements in technologies like quantum computing reveal fundamental limits. Current systems may not always produce the expected outcomes due to decoherence and noise. These challenges hinder deeper insights, especially in intricate phenomena such as superconductivity or the behavior of particles at extreme energies. Moreover, classical physics concepts often conflict with quantum principles, complicating how we understand these systems.
The Quest for Unified Theories
To overcome these limitations, the search for unified theories becomes vital. Physicists aim to merge quantum mechanics with general relativity, providing a comprehensive framework. This pursuitโs essence lies in simplifying complex interactions into coherent models.
String Theory
String theory represents one promising avenue. This model suggests that fundamental particles are not point-like objects but rather one-dimensional strings. The uniqueness of string theory lies in its ability to unify different forces under a single framework.
Although string theory remains largely theoretical, its attributes promote exploration. It also allows researchers to apply mathematics to various branches of physics, demonstrating its potential in unifying aspects of gravity and quantum mechanics. However, it has limitations too, primarily lacking experimental evidence thus far.
Quantum Gravity
Quantum gravity is another domain with significant implications. It seeks to describe how gravity functions at quantum scales. One of its primary characteristics is how it reconciles gravitational force with quantum mechanics. This is vital not only for theoretical integrity but also for practical applications in cosmology and particle physics.
The challenge lies in demonstrating quantum gravity principles through experiments. Some theoretical models, such as loop quantum gravity, offer insights but face skepticism because they too lack empirical validation. Still, they provide direction for theoretical advancements and encourage exploration.
Future Research Directions
As the scientific community confronts these obstacles, future research directions become essential. These paths offer opportunities to expand our understanding of energy quantization and its applications.
Experimentation and Observation
Experimentation and observation form the backbone of scientific progress. Advancements in technology present new tools for investigating quantum mechanics. Techniques such as high-energy particle colliders and advanced imaging allow for unprecedented observations in conducting experiments.
The key characteristic here is their potential to provide data that could validate or refute existing theories. New methods can lead to discoveries about energy states and behaviors not observed before. Invigorating research in this area helps bridge gaps in knowledge, offering hope for clarifying elusive concepts in quantum mechanics.
The Role of AI in Quantum Research
Artificial intelligence (AI) increasingly plays a role in advancing quantum research. Machine learning algorithms can analyze vast data sets rapidly, identifying patterns that human researchers may overlook. This capability is particularly beneficial in modeling complex quantum states and streamlining experimental designs.
AI's unique feature lies in its adaptability; it learns from data and improves over time. However, reliance on AI also has challenges, such as the need for accurate inputs and interpretations. Nevertheless, the integration of AI could revolutionize how researchers explore energy quantization.
Ending
The conclusion serves as a vital component of the article, encapsulating the fundamental aspects related to the concept of energy quanta. It reinforces the importance of understanding energy quantization within the broader framework of physics and its applications in various scientific fields. By summarizing the key insights presented throughout the text, this section aims to synthesize the information, offering a coherent overview that benefits students, researchers, educators, and professionals.
Energy quantization plays a critical role in our comprehension of both quantum mechanics and the universe. It offers different perspectives on traditional physics and sheds light on complex phenomena. Moreover, the implications of energy quanta extend beyond theoretical inquiry, impacting technological advancement and practical applications in diverse areas such as quantum technology and sustainability.
Summary of Key Insights
Understanding the quanta of energy unveils several pivotal insights:
- Fundamental Nature: Energy quantization is foundational to quantum mechanics, illustrating the discrete nature of energy levels.
- Technological Impact: Innovations in areas like quantum computing and energy harvesting technologies employ principles derived from energy quantization.
- Interdisciplinary Relevance: The relationship between quantum mechanics, chemistry, and biology demonstrates the pervasive influence of energy quanta on various scientific domains.
This study reveals that the implications of energy quantization are substantial, as they pave the way for groundbreaking research and understanding of the universe.
The Continuing Importance of Energy Quanta
The significance of energy quanta is enduring and multifaceted. As research progresses, the fundamental insights derived from this concept continue to shape our understanding.
- Advancements in Quantum Computing: Energy quanta underpin the functioning of quantum computers, which promise increased computational power and efficiency. This evolution has far-reaching implications for fields like cryptography and artificial intelligence.
- Sustainable Energy Solutions: Applications like photovoltaics rely on energy quantization for harnessing solar energy. This relevance points to the prospect of renewable resources in addressing climate change.
- Philosophical Implications: The exploration of energy quantization invites philosophical discussions regarding the nature of reality and our place in the universe, fostering a deeper appreciation for the complexity of the cosmos.