Mitochondria in Cells: The Powerhouse of Life
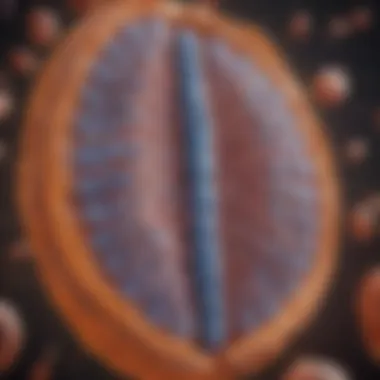
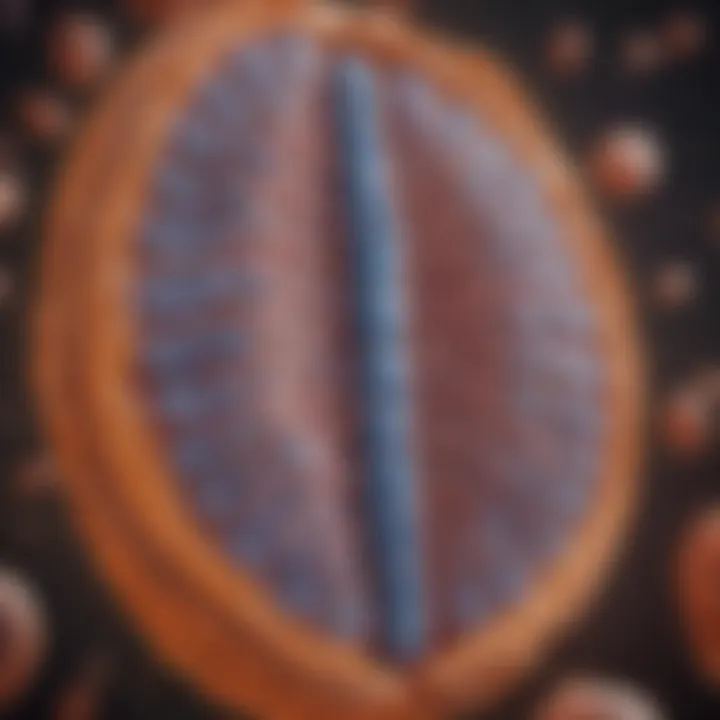
Intro
Mitochondria are essential organelles found in eukaryotic cells. They play a critical role in energy production, cellular metabolism, and overall cell health. Understanding the structure and function of mitochondria is crucial for comprehending how cells operate and how various diseases can impact human health. Recent research has shed light on the complexities of mitochondrial function and their genetic makeup. This article will explore these aspects and their implications for physiology and pathology.
Key Research Findings
Overview of Recent Discoveries
Recent studies have brought new insights into the role of mitochondria in cellular processes. One of the most significant findings is the relationship between mitochondrial dynamics and metabolic regulation. Mitochondria are not static; they can change shape, fuse together, or divide according to cellular needs. This adaptability is vital for maintaining cellular energy balance. Researchers have also identified specific mitochondrial proteins that influence how these organelles respond to stress and regenerate.
Another notable discovery involves the genetic makeup of mitochondria. Unlike nuclear DNA, mitochondrial DNA is inherited maternally and encodes essential proteins for the mitochondria's energy-producing machinery. Mutations in these genes can lead to various mitochondrial diseases, affecting muscle and brain function. Recent advancements in gene-editing technologies, such as CRISPR-Cas9, have opened new avenues for potential therapies targeting these genetic issues.
Significance of Findings in the Field
The findings related to mitochondrial dynamics and genetics greatly enhance our understanding of cellular biology. They suggest that mitochondrial health is not just a matter of energy production but also plays roles in cell signaling and regulating apoptosis, the process of programmed cell death.
New therapeutic strategies are emerging that aim to enhance mitochondrial function. For instance, pharmacological agents that target mitochondrial pathways are being studied for their potential in treating metabolic disorders and neurodegenerative diseases.
"Mitochondria are at the center of many biological processes, highlighting their significance beyond energy production."
Breakdown of Complex Concepts
Simplification of Advanced Theories
To further grasp mitochondrial function, it is helpful to simplify some advanced theories. Mitochondria convert nutrients into adenosine triphosphate (ATP), the energy currency of the cell. This process involves multiple stages, including glycolysis, the citric acid cycle, and oxidative phosphorylation.
- Glycolysis: Breakdown of glucose into pyruvate, producing ATP and NADH.
- Citric Acid Cycle: Further oxidation of pyruvate, yielding additional energy carriers like NADH and FAD.
- Oxidative Phosphorylation: The primary ATP production occurs within the electron transport chain, utilizing oxygen to produce water.
Visual Aids and Infographics
Using diagrams can also facilitate understanding. Infographics illustrating the stages of ATP production, as well as mitochondrial structure, can provide quick reference points for complex topics. These visual aids simplify information, aiding in better retention and comprehension for students, researchers, and professionals alike.
Prelims to Mitochondria
Mitochondria are critical components within eukaryotic cells, often referred to as the "powerhouses" of the cell. This is due to their primary role in generating adenosine triphosphate (ATP), the energy currency that fuels various cellular processes. Understanding mitochondria is essential for grasping how cells function, maintain homeostasis, and respond to differing physiological demands. This section serves as a foundational overview, setting the stage for a deeper exploration of their intricate structures and functions.
Definition and Importance
Mitochondria are double-membraned organelles present in nearly all eukaryotic cells. They convert biochemical energy from nutrients into ATP through cellular respiration. Mitochondria not only play a pivotal role in energy production but they also participate in metabolic pathways, apoptosis, and the regulation of cellular metabolism. Their ability to produce energy efficiently makes them indispensable for high-energy demanding tissues, such as muscle and nerve cells.
The importance of mitochondria reaches beyond just energy production. They are involved in heat generation, regulation of cellular metabolism, and maintenance of cellular health. Dysfunctional mitochondria can lead to a range of health issues, including metabolic disorders, neurodegenerative diseases, and certain types of cancer. Therefore, a comprehensive understanding of mitochondria is vital for educators, researchers, and healthcare professionals alike.
Historical Perspective
The concept of mitochondria dates back to the mid-19th century when scientists first observed these structures under a microscope. Initially, they were thought to be simple repositories of cellular energy. However, as research progressed, the role of mitochondria evolved into a complex understanding of their involvement in various cell functions.
The endosymbiotic theory, proposed in the 1960s by Lynn Margulis, suggested that mitochondria originated from free-living prokaryotic cells that entered into a symbiotic relationship with ancestral eukaryotic cells. This theory helped explain the presence of their own DNA, which is circular and resembles bacterial genomes.
Understanding mitochondrial history has allowed researchers to unravel their intricate workings and appreciate the evolutionary significance of these organelles. Such insights not only enhance our knowledge of cell biology but also pave the way for advancements in the study of diseases linked to mitochondrial dysfunction.
Structural Overview of Mitochondria
Understanding the structural components of mitochondria is essential for appreciating their diverse roles in cellular functions. The architecture of mitochondria is intricate and tailored for optimal performance in energy production, metabolism, and signaling. Each structural element contributes uniquely to the mitochondrion's ability to manage biochemical energy conversion and overall cellular health.
Mitochondrial Membranes
Outer Membrane
The outer membrane of mitochondria serves as a barrier between the cytoplasm and the mitochondrial interior. It is relatively permeable due to the presence of porins, which are integral membrane proteins that allow small molecules to pass through. This feature enables efficient transport of metabolites. The outer membrane also contains enzymes involved in lipid synthesis, making it vital for maintaining mitochondrial health and function. Its relatively fluid nature allows for integration with other cellular membranes, a key aspect for effective cellular interaction.
Inner Membrane
The inner membrane is distinct, highly convoluted, and enriched with proteins critical for electron transport and ATP synthesis. This membrane is impermeable to most ions and small molecules, creating a compartmentalized environment necessary for maintaining the proton gradient used in ATP production. The unique folding of this membrane forms structures known as cristae, which increase surface area, optimizing energy production processes. The inner membrane plays a crucial role in the organelle's bioenergetics.
Intermembrane Space
The intermembrane space exists between the outer and inner membranes. It plays a crucial role in cellular respiration by acting as a conduit for protons. When electrons move through the electron transport chain located in the inner membrane, protons are pumped into this space. This proton accumulation is critical for generating a proton gradient that drives ATP synthesis. The intermembrane space's composition and functions make it an important area for understanding how mitochondria execute energy metabolism effectively.
Mitochondrial Matrix
Enzymatic Functions
The mitochondrial matrix contains various enzymes responsible for metabolic processes, including the Krebs cycle and fatty acid oxidation. This compartment provides a favorable environment for these reactions, ensuring that nutrients are efficiently converted into ATP and other essential metabolites. The matrix's specialized conditions, including the presence of substrates and enzymatic cofactors, support the intricate metabolic pathways essential for cellular function.
Metabolic Pathways
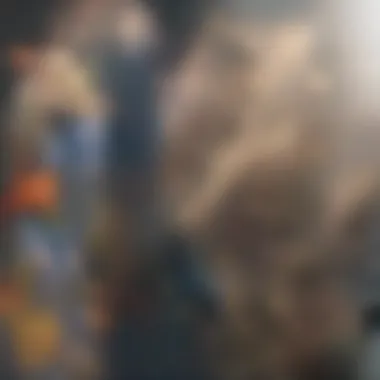
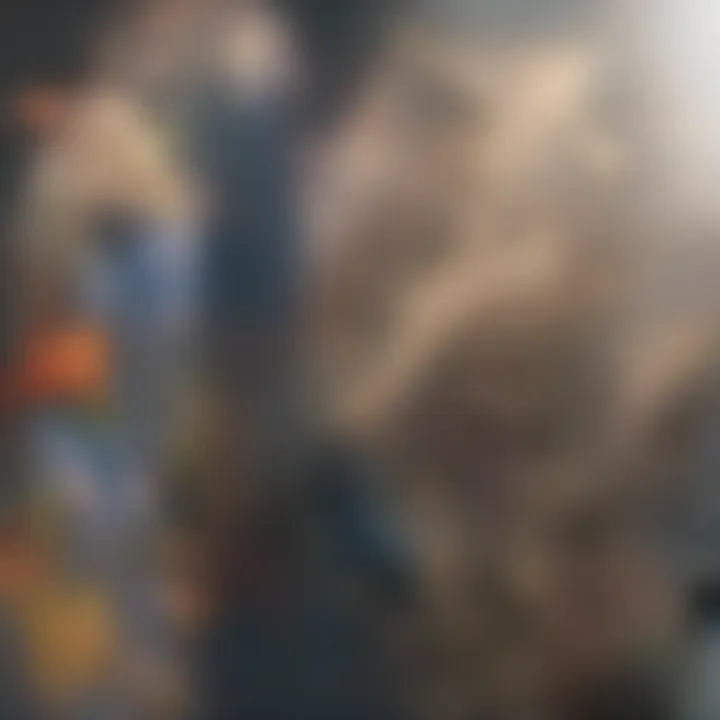
In addition to the Krebs cycle, the matrix is central to multiple metabolic pathways. It also plays a role in amino acid metabolism and the urea cycle. The strategic location of these pathways highlights the matrix's importance in overall metabolic balance. Effective functioning of the metabolic pathways within the matrix is fundamental for maintaining energy homeostasis and supporting cellular viability under various physiological conditions.
Cristae Structure
Formation and Function
Cristae are produced by the invagination of the inner membrane, dramatically increasing its surface area. This unique structure is significant for maximizing ATP synthesis capabilities. The formation of cristae is not only crucial for energy production but also allows for localized metabolic processes, which enhance efficiency at the cellular level. Dysfunction in cristae formation can impact mitochondrial function and overall energy homeostasis.
Role in ATP Synthesis
The cristae structure is integral to ATP synthesis, as it houses integral enzymes of the electron transport chain. Their arrangement and the surface area they provide optimize the coupling of electron transport with ATP production through oxidative phosphorylation. The arrangement of cristae also influences the efficiency of energy conversion processes. Thus, understanding cristae is fundamental to grasping the energetics of mitochondria and their relevance in health and disease.
Mitochondrial Functions
Mitochondria serve multiple pivotal functions essential for cellular health and energy management. Their capabilities extend beyond ATP production; they are involved in various metabolic processes that sustain life. Understanding mitochondrial functions helps in deciphering cellular responses to environmental changes and their implication in different diseases.
Role in ATP Production
Oxidative Phosphorylation
Oxidative phosphorylation is a crucial process that generates ATP, the energy currency of cells. This process occurs in the inner mitochondrial membrane and relies on the electron transport chain. Electrons derived from nutrients are transferred through a series of protein complexes, resulting in the pumping of protons into the intermembrane space. This creates a proton gradient.
A key characteristic of oxidative phosphorylation is its efficiency in ATP synthesis. Compared to other energy-producing methods, it yields a significantly higher number of ATP molecules per glucose molecule metabolized. The unique feature of this process is the coupling of electron transport to ATP synthesis, mainly through the action of ATP synthase, which utilizes the proton gradient to convert ADP and inorganic phosphate into ATP. The major advantage of oxidative phosphorylation is its high energy yield, making it a central aspect of cellular metabolism.
Chemiosmotic Coupling
Chemiosmotic coupling complements oxidative phosphorylation by linking the energy from electron transport to ATP production. The flow of protons back into the mitochondrial matrix is facilitated by the ATP synthase enzyme, capitalizing on the previously established proton gradient.
The essential aspect of chemiosmotic coupling is its reliance on the proton motive force. This mechanism is instrumental for efficient ATP generation. Furthermore, it illustrates the intimate connection between electron transport and ATP production. As an advantage, chemiosmotic coupling is a universal mechanism present in various organisms, underscoring its importance in energy metabolism.
Metabolism of Nutrients
Fatty Acid Oxidation
Fatty acid oxidation represents a fundamental aspect of mitochondrial function, primarily responsible for metabolizing lipids to produce energy. Through a series of enzymatic reactions, fatty acids are broken down into acetyl-CoA, which enters the citric acid cycle and ultimately participates in ATP generation.
The unique characteristic of fatty acid oxidation is its ability to supply a substantial energy source, especially during fasting or prolonged exercise. The oxidation of fatty acids yields more ATP than glucose, making it a favorable pathway during energy-deprived states. However, one downside is its inefficient utilization in some tissues that prefer carbohydrates, showing the context-dependent nature of energy metabolism.
Amino Acid Metabolism
Amino acid metabolism in mitochondria serves as another inlet into energy production and synthesis of important biomolecules. The mitochondria can deaminate amino acids, yielding intermediates that enter the citric acid cycle.
This process is significant as it demonstrates the versatility of mitochondrial functions. Amino acids not only provide substrates for energy but are also crucial for the biosynthesis of proteins and other nitrogenous compounds. One aspect to consider is that excessive amino acid catabolism can lead to imbalances, potentially affecting overall metabolic health by producing toxic ammonia. This dual aspect makes it a vital area of study.
Regulation of Metabolism
Metabolic Flexibility
Metabolic flexibility refers to the ability of cells to adapt fuel utilization based on availability and energy demands. Mitochondria play a critical role in this, capable of switching between carbohydrate and fat oxidation depending on the physiological conditions.
A significant feature of metabolic flexibility is its contribution to maintaining homeostasis. This adaptability is especially important during various metabolic states, such as exercise or fasting. However, a lack of metabolic flexibility is linked to metabolic disorders, indicating its importance in health and disease.
Response to Energy Demands
The response to energy demands highlights how mitochondria adjust their activity in relation to cellular energy needs. Mitochondrial biogenesis can increase energy supply through the synthesis of more mitochondria, especially during high energy-requirement scenarios like physical activity.
The key characteristic of this response is its dynamic nature. Mitochondria can rapidly adapt to changes in energy demand by modulating their functional capacity. On the downside, chronic overworking of mitochondria can result in stress, contributing to various pathologies. Proper balance is essential to ensure cellular health.
Mitochondrial Genetics
Mitochondrial genetics is central to understanding the complex roles mitochondria play in cellular function. The unique genetic structure and inheritance patterns of mitochondria distinguish them from nuclear DNA. Each aspect of mitochondrial DNA (mtDNA) holds significance for cell biology, genetic inheritance, and pathogenic studies. These genetic characteristics provide insights into how energy production is closely linked to genetic stability and health.
Mitochondrial DNA Structure
Mitochondrial DNA is a small circular DNA molecule. Its structure consists of approximately 16,500 base pairs, encoding 37 genes, which are involved primarily in energy production and mitochondrial function. Unlike nuclear DNA, mtDNA lacks introns and is highly efficient. This streamlined structure allows rapid replication and expression, making it essential for maintaining mitochondrial function. Each mitochondrion can contain multiple copies of this DNA, ensuring that the cell can produce sufficient energy.
Inheritance Patterns
Genetic inheritance of mtDNA follows a distinct pattern. Mitochondrial DNA is typically inherited maternally. This phenomenon occurs because, during fertilization, the sperm contributes minimal cytoplasm, while the egg provides the majority of cellular material. Thus, all offspring inherit their mitochondrial genome from their mother. This aspect of mitochondrial inheritance is a key point in genetic studies, allowing researchers to track lineage and genetic disorders through maternal lines.
Maternally Inherited Genome
The maternally inherited genome primarily influences studies related to population genetics and evolutionary biology. One notable characteristic of this inheritance pattern is its lack of recombination. This leads to high conservation of mitochondrial genes across generations, making it easier for scientists to trace evolutionary events. However, this approach also limits genetic diversity. The maternal lineage can provide critical insights into human migration and population history.
Implications for Genetic Studies
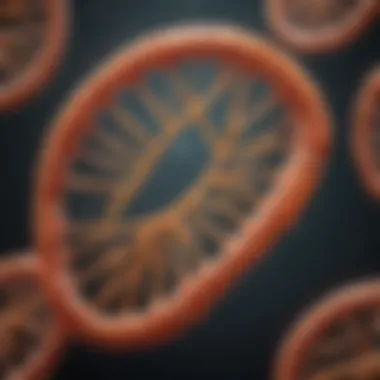
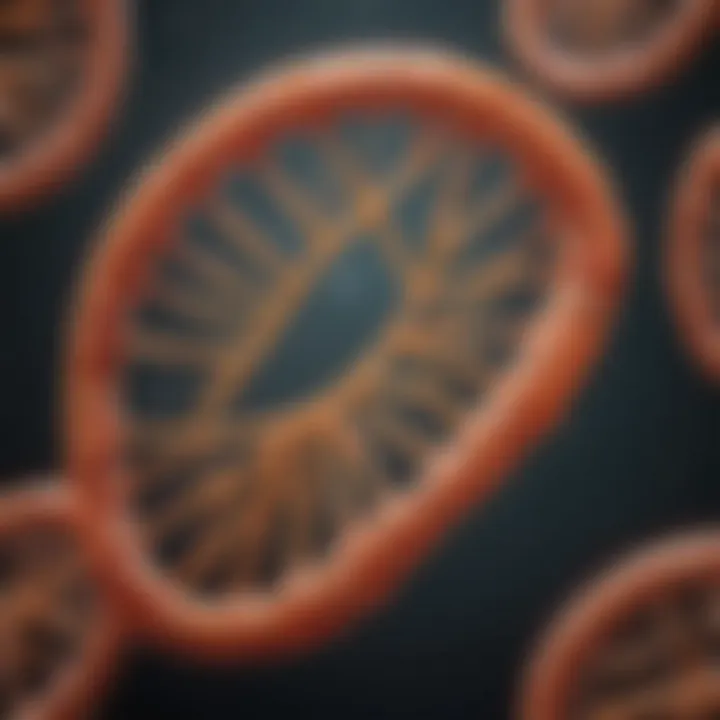
The implications for genetic studies lie in the ability to explore disease associations through maternal lineage. Due to its conserved nature, mtDNA variations can signal historical and biological significance. The primary characteristic that makes this beneficial is the connection of certain mtDNA mutations to specific diseases, allowing for potent diagnostic markers. Understanding these patterns assists researchers in developing therapeutic strategies targeting mitochondrial dysfunction.
Mutations and Their Effects
Mutations in mitochondrial DNA can profoundly affect mitochondrial function and overall cell health. These mutations can be classified into various types, each with different implications for cellular physiology.
Types of Mutations
Common types of mutations include point mutations, deletions, and insertions. Point mutations in mtDNA may lead to changes in amino acid sequences, potentially disrupting protein function. Deletion mutations result in loss of essential genes, which can severely impair respiratory chain function. These variations are particularly impactful as they can affect energy metabolism within cells, making them crucial for understanding mitochondrial diseases.
Disease Associations
Mitochondrial mutations are linked to a growing number of diseases, including mitochondrial myopathy and neurodegenerative disorders. The key characteristic of these associations is the role of energy metabolism impairments in disease pathogenesis. Many mitochondrial DNA mutations have been identified as key contributors to diseases. Studying these correlations helps in identifying at-risk populations and developing targeted therapies. However, the complexity of these diseases often involves multifactorial influences, complicating the development of interventions.
Mitochondrial Dysfunction and Disease
Mitochondrial dysfunction plays a critical role in various diseases, making this topic particularly important in understanding cellular health. Mitochondria are essential not just for energy production but also for maintaining cellular homeostasis. When they fail to function properly, it can lead to significant metabolic issues. The study of mitochondrial dysfunction offers insights into a range of conditions, from metabolic disorders to degenerative diseases.
Pathophysiology
Mitochondrial dysfunction can manifest in numerous ways and is associated with various pathophysiological conditions. Identifying these conditions can provide a better understanding of their complexities.
Metabolic Disorders
Metabolic disorders linked to mitochondrial dysfunction involve alterations in energy metabolism. Many patients exhibit symptoms, including fatigue, weakness, and exercise intolerance. One key characteristic of metabolic disorders is their heterogeneity, stemming from genetic factors that differ even among patients with similar presentations. This makes their study crucial for a comprehensive understanding of energy metabolism. Their unique feature often includes abnormal metabolite accumulation, which can help in diagnosis. Understanding these disorders aids in tailoring specific treatments, although challenges remain due to the complexity of metabolic pathways.
Neurological Implications
Mitochondrial dysfunction has notable neurological implications. Conditions such as Parkinsonβs disease and Alzheimerβs disease often trace their origins to compromised mitochondrial function. A significant characteristic of these implications is the focus on how energy deficits in neurons lead to cell death and cognitive decline. The study of neurological implications is beneficial for recognizing early signs of age-related cognitive decline, providing crucial data for intervention strategies. Unique features involve synaptic dysfunction and increased oxidative stress, presenting both diagnostic opportunities and therapeutic challenges, especially in regard to neuroprotection strategies.
Role in Aging
Aging is inherently linked to mitochondrial function. The decline in mitochondrial efficiency correlates with age-related diseases and overall health decline.
Oxidative Stress
Oxidative stress arises when there is an imbalance between reactive oxygen species and antioxidant defenses. It is a core aspect of how mitochondrial dysfunction affects aging. A key characteristic is that as mitochondria age, they produce more reactive oxygen species, leading to increased cellular damage. This concept is particularly relevant as it highlights the need for antioxidants in health and longevity studies. Unique features include the impact of lifestyle factors on oxidative stress, illustrating that interventions such as diet and exercise can modify effects over time. This has both advantages and disadvantages in developing effective age-related therapies, as results can vary based on individual responses.
Mitochondrial Biogenesis
Mitochondrial biogenesis refers to the process by which cells increase their mitochondrial mass and function. This is relevant for maintaining energy homeostasis in the face of stressors. The ability to adapt is a key characteristic of mitochondrial biogenesis. This adaptability is particularly beneficial in understanding how exercise may promote mitochondrial growth and improve overall cellular health. Unique factors such as the role of PGC-1Ξ± in regulating biogenesis can serve as a target for therapeutics, highlighting both opportunities and challenges in translating findings into clinical settings.
Mitochondria in Cancer
Mitochondrial function is also crucial in understanding cancer biology. Cancer cells often display altered metabolism, which can be directly traced to mitochondrial activity.
Energy Metabolism in Cancer Cells
Energy metabolism in cancer cells is markedly different from that in normal cells. Tumor cells frequently rely on glycolysis, even in the presence of oxygen, a phenomenon known as the Warburg effect. This key characteristic allows them to grow rapidly and survive in adverse conditions. Understanding this shift is beneficial for developing targeted treatments. Unique features include the potential to target the altered metabolic pathways with specific inhibitors, although challenges arise from the adaptability of cancer cells and their resistance mechanisms.
Therapeutic Implications
The therapeutic implications of targeting mitochondrial function in cancers are profound. Drugs that affect mitochondrial dynamics could help to selectively induce apoptosis in cancer cells. A notable characteristic of these approaches is the suggestion that they can complement traditional therapies. This is beneficial for improving outcomes and reducing side effects in patients. However, unique features include the variability in patient responses and the need for personalized approaches in treatment planning, highlighting both the promise and limitations of current research.
Mitochondrial Biogenesis
Mitochondrial biogenesis is a crucial process for maintaining cellular energy homeostasis and overall cellular health. The organelles are not only the powerhouses for ATP production but are also dynamic structures that adapt to the metabolic needs of cells. Understanding the mechanisms that regulate mitochondrial biogenesis can provide insight into various physiological and pathological conditions, making this topic essential in the study of cellular biology. This section will discuss the regulatory mechanisms and stimuli that influence mitochondrial biogenesis, highlighting their significance in health and disease.
Regulatory Mechanisms
Transcriptional Control
Transcriptional control plays a vital role in regulating mitochondrial biogenesis by modulating the expression of genes related to mitochondrial function. A key characteristic of transcriptional control is its ability to respond to cellular energy demands. It allows cells to increase the production of mitochondrial components when energy production needs to rise. This mechanism is beneficial for maintaining energy homeostasis, especially in muscle and brain tissues, which have high energy requirements.
One unique feature of transcriptional control is the synergistic action of transcription factors such as PGC-1Ξ± and NRF1. These factors coordinate the expression of nuclear and mitochondrial genes, optimizing mitochondrial function. However, one drawback is that transcriptional control can be affected by various factors, such as aging and disease, which may lead to impaired mitochondrial function and contribute to conditions like metabolic syndrome.
Post-translational Modifications
Post-translational modifications (PTMs) also significantly contribute to mitochondrial biogenesis. They involve the chemical modification of proteins after translation, influencing their activity, localization, or stability. A key characteristic of PTMs is their ability to provide rapid responses to environmental changes. This allows for a more immediate remodeling of mitochondrial activities, which is advantageous with changing metabolic demands.
One notable feature of PTMs is the role of acetylation and phosphorylation in controlling the activity of mitochondrial proteins. These modifications can enhance or inhibit mitochondrial functions in a dynamic manner. However, the complexity of PTMs can make it challenging to understand how multiple modifications might interact, potentially leading to dysregulated mitochondrial function in certain diseases.
Stimuli and Adaptation
Exercise and Physical Activity
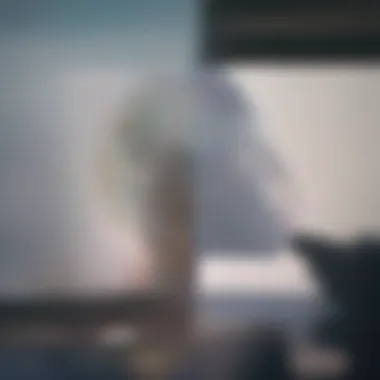
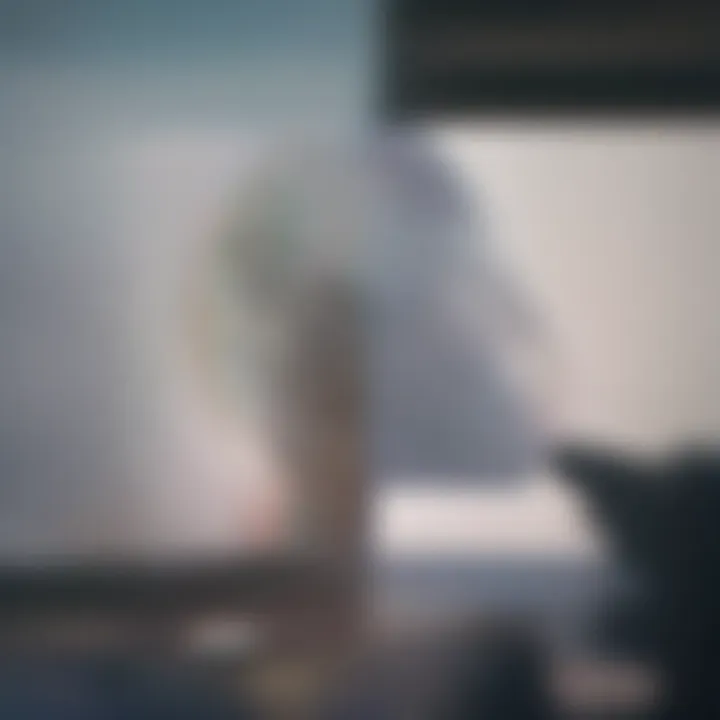
Exercise and physical activity serve as powerful stimuli for mitochondrial biogenesis. Physical activity induces metabolic stress that can signal cells to increase mitochondrial content and function. The primary benefit here is the enhancement of energy production capacity, which is crucial for maintaining performance during prolonged physical efforts. Regular exercise is popularly recognized for its positive effects on mitochondrial function, which translates to improved overall health.
The unique aspect of this adaptation is that it not only affects existing mitochondria but also promotes the generation of new ones, enhancing the overall mitochondrial network. However, excessive exercise without proper recovery might lead to mitochondrial overload and result in fatigue or injury, illustrating the need for balanced activity.
Caloric Restriction
Caloric restriction (CR) has been shown to influence mitochondrial biogenesis through enhanced metabolic signaling pathways. One of the key characteristics of caloric restriction is its ability to regulate cellular energy levels, promoting a shift towards energy efficiency. This approach is beneficial, especially in the context of longevity and metabolic health, as it encourages the optimization of mitochondrial function and reduces the risk of age-related diseases.
A unique feature of caloric restriction is its impact on sirtuins, proteins that promote mitochondrial biogenesis. They enhance cellular stress responses and play a role in metabolism. However, prolonged caloric restriction, if not undertaken carefully, can lead to nutrient deficiencies and potential negative health effects, underlining the importance of understanding its role in mitochondrial biogenesis.
Mitochondrial biogenesis is a complex interplay of genetic, environmental, and lifestyle factors. This understanding is vital for developing strategies to enhance mitochondrial health and combat diseases.
In summary, mitochondrial biogenesis serves essential functions in cellular energy management and adaptation to environmental stresses. Regulatory mechanisms like transcriptional control and post-translational modifications, along with stimuli like exercise and caloric restriction, highlight the complexity and necessity of maintaining robust mitochondrial function.
Therapeutic Approaches Targeting Mitochondria
The exploration of therapeutic approaches that target mitochondria has gained traction in recent years. This focus on mitochondria is significant, given their central role in energy production and cellular metabolism. Mitochondrial dysfunction is linked to a variety of diseases including neurodegenerative disorders, diabetes, and cancer. Therefore, finding methods to manipulate mitochondrial function holds promise for novel treatment strategies.
Mitochondrial Pharmacology
Targeted Drug Delivery
Targeted drug delivery is an essential component of mitochondrial pharmacology. It aims to deliver therapeutic agents directly to mitochondria, minimizing side effects and maximizing efficacy. The key characteristic of this approach is specificity; drugs are designed or modified so they can be recognized and taken up by mitochondria specifically. This is particularly advantageous because many compounds, if delivered systemically, may not reach the mitochondria effectively.
One unique feature of targeted drug delivery is the usage of ligands that bind selectively to mitochondrial membrane proteins. This enhances the concentration of drugs at the site of action, which improves therapeutic outcomes. However, this method also faces challenges, including the complexity of drug formulation and potential issues in clinical implementation.
Small Molecule Activators
Small molecule activators serve as another key strategy in targeting mitochondria. These compounds can enhance mitochondrial function by improving processes such as ATP production or by reducing oxidative stress. The notable characteristic of small molecule activators is their ability to penetrate cellular membranes easily, enabling them to reach mitochondria with relative ease.
Their unique feature lies in their versatility; these compounds can modulate various mitochondrial pathways. One advantage of small molecule activators is their potential to offer quick and potent responses in cellular contexts. However, their actions may also lead to off-target effects or dose-dependent toxicity, which must be carefully considered during development.
Gene Therapy
Foreword to Gene Editing
Gene editing has emerged as a promising approach to correct mitochondrial dysfunction at the genetic level. This technique allows precise alterations in mitochondrial DNA and can potentially reverse deleterious mutations. The core aspect of gene editing is its precision, enabling researchers to target specific genes responsible for mitochondrial diseases. This precision is a strong point for gene therapy, making it a beneficial area of focus in mitochondrial research.
A unique feature of gene editing methods, like CRISPR-Cas9, is their adaptability. They can be customized to target various mitochondrial genes effectively. However, this approach faces significant challenges, including ethical considerations and the technical difficulty of delivering editing tools specifically to mitochondria.
Potential Applications in Mitochondrial Disease
The potential applications of gene therapy in mitochondrial disease are vast. By correcting mutations in mitochondrial DNA, there is the possibility of stopping the progression of disease and restoring mitochondrial function. The main characteristic of this application is its ability to address the root cause of mitochondrial disorders rather than just managing symptoms.
A unique feature of these applications is their focus on rare, inherited diseases, which currently lack effective treatments. While the promise is substantial, issues related to gene delivery techniques and patient safety remain critical considerations that need to be addressed before these therapies can be widely used in clinical practices.
Therapeutic strategies targeting mitochondria provide hope for innovative treatments, but ongoing research is essential to unravel the complexities of these approaches and their potential implications.
Future Directions in Mitochondrial Research
Research on mitochondria has expanded significantly over recent years, revealing their central role in various cellular processes. Future directions in this field hold great importance as researchers aim to uncover new insights into mitochondrial function and dysfunction. Understanding these avenues can lead to improved diagnostic and therapeutic approaches to diseases linked to mitochondrial dysfunction.
Advancements in Imaging Techniques
Real-Time Monitoring
Real-time monitoring represents a crucial advancement in the study of mitochondrial dynamics. This technique enables researchers to observe mitochondrial behavior in live cells, providing immediate feedback on their functionality. The ability to track real-time changes enhances our understanding of mitochondrial reactions to various stimuli and medications.
One of the key characteristics of real-time monitoring is its capacity to visualize changes over time, offering more contextual information than static images. Moreover, it is a widely favored approach due to its efficiency in collecting data without affecting cell viability. However, one challenge is ensuring the accuracy of the measurements under varying conditions. The use of sensitive fluorescent indicators plays a unique role, allowing for precise detection of mitochondrial parameters like membrane potential and metabolic activity.
High-Resolution Imaging
High-resolution imaging has revolutionized our ability to observe mitochondrial structures at the nanoscale. This technique allows scientists to access fine details of mitochondrial morphology and spatial organization. Understanding these attributes can lead to new insights into how mitochondrial structure correlates with their function.
The primary characteristic of high-resolution imaging is its power to depict small-scale structures, facilitating the study of cristae formation and other intricate details. Its popularity stems from the ability to address complications that arise from low-resolution techniques. Still, the significant cost and the requirement for specialized equipment can limit its accessibility for some research teams.
Integration with Artificial Intelligence
Data Analysis
Data analysis, leveraging artificial intelligence, plays a pivotal role in enhancing mitochondrial research outcomes. By utilizing advanced algorithms, researchers can analyze large datasets related to mitochondrial function more effectively. This method significantly accelerates the identification of patterns and anomalies that may be present within the collected data.
A key feature of AI-driven data analysis is its capacity to process vast amounts of information rapidly, enabling researchers to derive conclusions that would otherwise take much longer using traditional methods. It provides an efficient and dynamic approach, making it popular in the scientific community. However, one must consider that the accuracy of AI predictions relies heavily on the quality of the input data, necessitating rigorous pre-processing and validation steps.
Predictive Modeling
Predictive modeling is another integral aspect of AI integration in mitochondrial research. It allows scientists to create models that anticipate how mitochondria will respond to various genetic or environmental changes. These models can be instrumental in hypothesizing potential therapeutic interventions.
The primary characteristic of predictive modeling is its ability to simulate various scenarios using existing data. This approach is beneficial as it can save both time and resources by narrowing down experimental paths. Nonetheless, a significant challenge lies in ensuring that the models accurately reflect biological realities, as oversimplification can lead to misleading conclusions.
The future of mitochondrial research is set to be transformative, integrating innovative imaging techniques and advanced analytical methodologies to unveil the complexities of these essential organelles.