In-Depth Analysis of Thermodynamic Concepts
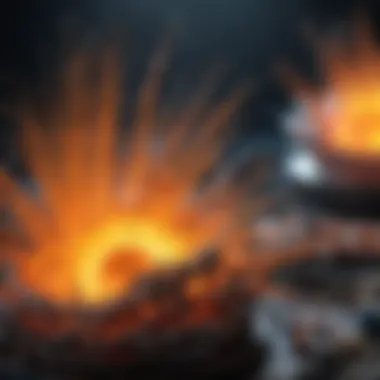
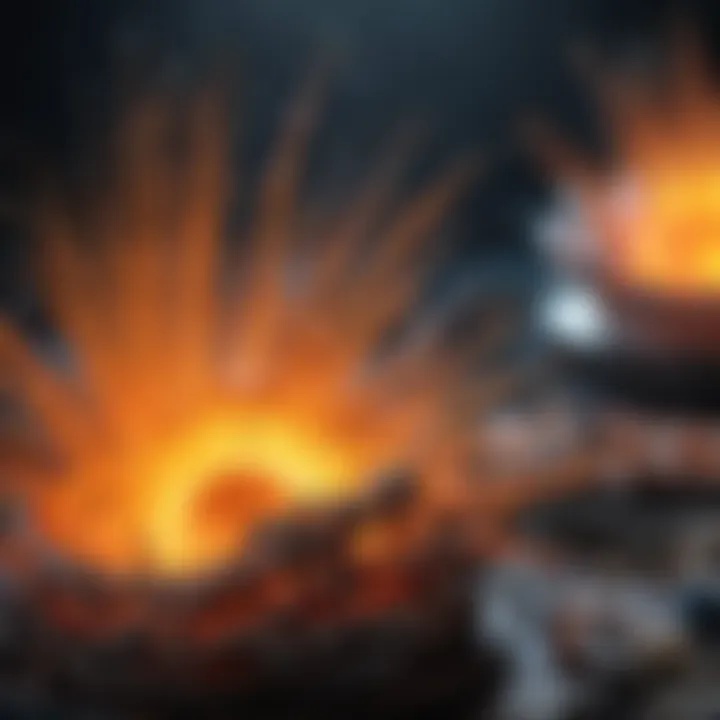
Intro
In the vast landscape of science, thermodynamics stands out as a cornerstone, deeply embedded in both theoretical and applied physics. Whether it’s the engine in your car or understanding the heat exchange processes in the atmosphere, thermodynamics plays a vital role. It’s not just an abstract concept relegated to dusty textbooks; it’s a vibrant field with real-world implications that touch nearly every corner of modern life.
As we embark on this exploration, we will unpack the intricate definitions that form the foundation of thermodynamic laws. From the historical evolution that shaped our understanding to the modern interpretations that guide current research and innovations, this article aims to present thermodynamics with clarity and depth. Let’s start to uncover the essence of this discipline, laying out the pieces that make up the grand puzzle of heat, energy, and work.
Key Research Findings
Overview of Recent Discoveries
Recent advances in thermodynamics have paved the way for exciting discoveries that bridge the gap between classical concepts and contemporary issues. Researchers have been focusing on the nexus of thermodynamics and information theory, revealing that the laws governing the flow of energy are closely related to the principles of information processing. This marriage of disciplines has led to insights on how systems can be optimized for efficiency.
Moreover, the rise of quantum thermodynamics introduces a fresh perspective, examining the behavior of heat and energy at the quantum level. This field is reshaping our understanding of microscopic systems, where particles behave in ways that defy classical intuition. The implications of such discoveries are profound, influencing how we approach energy management in technologies like quantum computing and nanotechnology.
Significance of Findings in the Field
The significance of these findings cannot be overstated. They enhance our grasp of energy transformation processes, which are pivotal in areas like renewable energy systems, materials science, and computational technology. Understanding the principles of thermodynamics in the context of these new discoveries helps researchers and practitioners create frameworks for innovation in energy-efficient designs and sustainable practices.
"The study of thermodynamics isn't just about heat; it's about understanding the fundamental rules that govern energy flow and transformation in our universe."
Breakdown of Complex Concepts
Simplification of Advanced Theories
Thermodynamics often appears daunting, laden with jargon and complex equations. To make these ideas more accessible, we can break them into fundamental components:
- Systems and Surroundings: Every thermodynamic analysis begins with defining a system (the part of the universe being studied) and its surroundings (everything else). This distinction is crucial for understanding energy exchanges.
- State Functions: Properties like pressure, volume, and temperature are state functions; they describe the state of a system at a given point. Navigating these functions helps simplify calculations and conceptual understanding.
- Energy Transformations: The heart of thermodynamics is energy transformations, typically between heat and work. Grasping how these transformations occur is vital for applications in engineering and environmental science.
Visual Aids and Infographics
To enhance the understanding of these complex concepts, visual aids can be instrumental. Diagrams illustrating cycles, such as the Carnot cycle or the refrigeration cycle, can provide instant clarity on how energy moves and transforms.
- Cycle Diagrams: These can show working processes and help visualize how energy input, output, and efficiency change.
- Graphs: Temperature-entropy (T-S) and enthalpy-entropy (H-S) plots can depict changes during thermodynamic processes, providing insight into efficiency and effectiveness.
In summary, thermodynamics, while intricate, can be made comprehensible through careful breakdown and engaging visuals. As we continue this exploration, we will delve deeper into the historical evolution and the contemporary definitions that unify these concepts into a coherent framework.
Prolusion to Thermodynamics
Thermodynamics stands as a cornerstone of physical sciences, intricately weaving together concepts that span the fields of physics, chemistry, biology, and engineering. Understanding thermodynamics is crucial, as it not only provides insights into energy transformations but also influences various technological advancements and natural phenomena. In this article, we embark on a thorough exploration of thermodynamics, aiming to elucidate its definitions, principles, and wide-reaching applications.
Definition of Thermodynamics
At its core, thermodynamics is the study of heat and temperature, and their relation to energy and work. It explores how energy moves within a system and how it changes, affecting the state and behavior of matter. The hallmark of thermodynamics lies in its ability to define systems, and the interactions that occur within and outside these systems.
For instance, consider the combustion of coal in a power plant. The coal, undergoing thermodynamic processes, transforms its chemical energy into heat, which then drives steam turbines, ultimately producing electricity. Here, thermodynamics not only explains what happens during combustion but also quantifies the energy transferred in forms like heat and work.
"Thermodynamics is a bridge between the macroscopic properties of materials and the microscopic behavior of their constituent particles."
A comprehensive grasp of thermodynamics encompasses understanding various terms and their significance. Key definitions often include:
- System: A defined portion of the universe under study.
- Surroundings: Everything outside the system.
- State Function: A property whose value depends only on the state of the system, not on how it arrived at that state.
Understanding these terms forms the bedrock of many principles in thermodynamics.
Historical Context of Thermodynamic Definitions
The evolution of thermodynamic definitions is like a tapestry woven by many scientists through centuries. The journey begins in the early 19th century when foundational ideas were established.
One notable figure is Sadi Carnot, who, in 1824, published Reflections on the Motive Power of Fire, laying the groundwork for the heat engine concept. This spark led to further refinements, mainly by scientists like Rudolf Clausius and Lord Kelvin, who developed the laws of thermodynamics in the latter half of the century.
Initially, thermodynamics was heavily influenced by steam engines and their efficiencies, which united mechanical engineering with physical sciences. As society shifted towards more complex systems—like automobiles and refrigerators—definitions evolved to address phenomena such as:
- The transformation of heat to work in engines.
- Equilibrium states in various processes.
- The limitations imposed by the second law of thermodynamics related to entropy.
Philosophical debates also emerged regarding the nature of heat and energy, spurring discussions around the conservation of energy and the inevitable increase of entropy in closed systems.
By the late 1800s, thermodynamics had transformed from a local curiosity of engineers to a critical discipline that underpins modern science and technology. Today, terms and definitions continue to be refined, shaped by ongoing research in fields as diverse as chemical engineering, astrophysics, and materials science.
In understanding these historical contexts, readers gain a richer perspective on how thermodynamics not only defines energy interactions but also narrates the story of scientific progress itself.
Core Concepts in Thermodynamics
Thermodynamics encompasses a series of fundamental principles that govern the interactions of energy and matter. Understanding core concepts not only provides clarity but also facilitates grasping advanced applications in varied fields such as engineering and physics. The core concepts serve as the backbone of thermodynamic laws, setting the stage for comprehending more complex interactions within thermodynamic systems.
Understanding the distinctions among systems and surroundings, state functions, and the conditions of thermodynamic equilibrium is crucial. Each aspect builds on the others, creating a comprehensive framework that researchers, educators, and students rely upon.
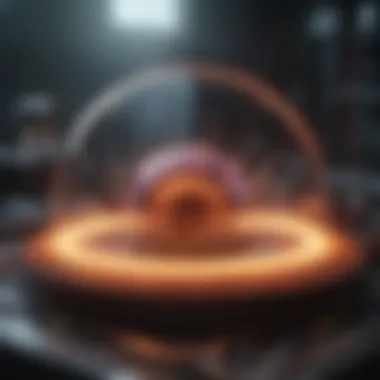
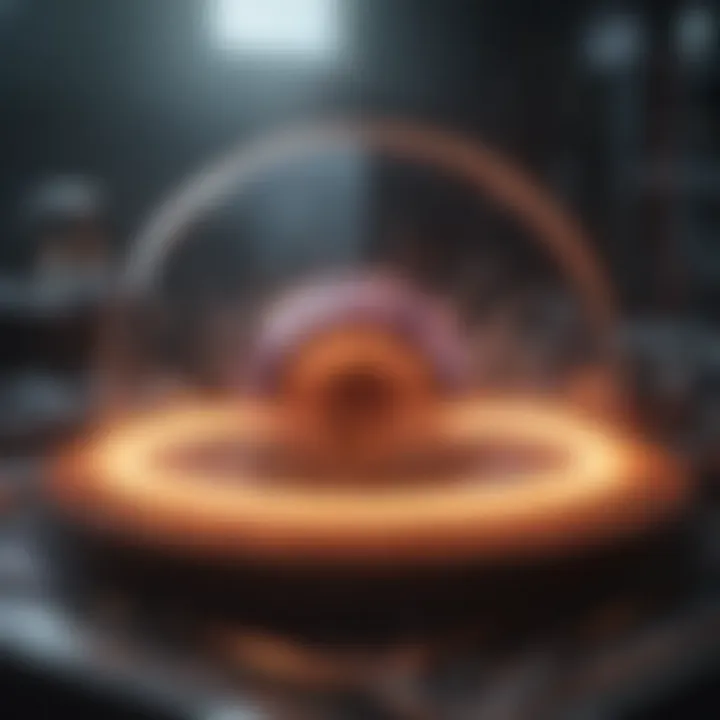
Systems and Surroundings
The distinction between systems and surroundings is foundational in thermodynamic studies, creating a structure within which we analyze energy transfer and transformation.
Closed Systems
Closed systems allow for energy transfer but prevent mass exchange with the surroundings. One defining characteristic of closed systems is their ability to maintain a consistent internal environment, which makes them a focal point in many theoretical and practical applications. For example, consider a steam boiler: it enables heat transfer while keeping the steam from escaping. This characteristic is beneficial as it eases analysis, yielding predictable behaviors based on the first law of thermodynamics, which speaks to energy conservation.
A unique feature of closed systems is their capacity to be modeled mathematically with relative ease. However, one disadvantage can be the oversimplification of real-world processes, where mass exchange can significantly affect outcomes.
Open Systems
Open systems, on the other hand, permit both energy and mass exchange with the surroundings. This aspect makes them particularly relevant for real-world applications such as engines or ecosystems. Their key characteristic of interaction with the environment allows for a more dynamic understanding of thermodynamic processes.
These systems are favored in studies involving biochemical processes, like cellular respiration, since they closely mirror actual biological functions. Moreover, their unique feature lies in the complexity of modeling, as numerous external variables must be accounted for. The main disadvantage, however, is the increased difficulty in isolating variables for rigorous analysis.
Isolated Systems
Isolated systems are those that do not exchange either mass or energy with their surroundings. This rare type of system resembles the Universe in theoretical discourse, making it an intriguing subject. The key characteristic here is complete insulation, which allows these systems to be seen as idealized environments for certain theoretical explorations.
Their benefit stems from the ability to simplify thermodynamic equations. However, the main drawback is that isolated systems rarely, if ever, occur in the real world; thus, they may not hold as much practical relevance.
State Functions
State functions are vital in thermodynamics because they describe the state of a system at equilibrium without considering the path taken to reach that state. The ability to pinpoint those functions is essential for analyzing energy transfer methods.
Internal Energy
Internal energy refers to the total energy contained within a system, accounting for both kinetic and potential forms. The key characteristic of internal energy is its dependence solely on the state of the system rather than on how it was reached. This makes it a crucial aspect for calculations pertaining to the first law of thermodynamics.
Being a beneficial choice for discussions around work and heat during processes, internal energy serves as a bedrock concept that aids in understanding energy balance in thermodynamic cycles. A unique feature is also its straightforward application in practical scenarios, although its main downside is that it cannot be measured directly; one can only determine changes in internal energy.
Enthalpy
Enthalpy is a measurement of the total heat content of a system, incorporating internal energy and the product of pressure and volume. Its key characteristic is its focus on constant pressure systems, making it particularly useful in engineering contexts, such as when designing heat exchangers and reactors.
The advantage of enthalpy is that it allows for easier calculations for processes where pressure remains constant, which is common in many industrial processes. However, it requires assumptions that might not always hold in real situations.
Entropy
Entropy is a measure of disorder or randomness in a system, serving as a lynchpin in understanding thermodynamic processes. One key characteristic of entropy is its tendency to increase over time, in accordance with the second law of thermodynamics, establishing a fundamental direction for energy transformations.
Its importance in this article arises from its implications for efficiency in real-world systems. A unique feature of entropy is its probabilistic nature; it provides insights into irreversible processes. While entropy has profound theoretical importance, it can pose challenges in practical calculations, as measuring absolute entropy is often indirect.
Thermodynamic Equilibrium
Thermodynamic equilibrium represents a state in which macroscopic properties of a system are unchanging over time. This condition facilitates meaningful discussion of systems since the rates of all processes in the system have reached a balance. Its importance cannot be understated: without equilibrium, many properties and laws cannot be adequately applied.
Recognizing these core concepts enables deeper insights into the laws of thermodynamics, paving the way for their applications across diverse fields.
The Laws of Thermodynamics
The laws of thermodynamics form the backbone of this scientific discipline, as they encapsulate the fundamental principles governing energy transfer and conversion. Understanding these laws is crucial as they provide insight into how energy flows within various systems and relate directly to real-world phenomena. Notably, each law builds upon the others, creating a comprehensive framework that applies across numerous fields such as physics, chemistry, and engineering. This interconnectedness not only enhances our understanding of the universe but also allows for innovations in technology and sustainable practices.
Zeroth Law of Thermodynamics
The Zeroth Law establishes the concept of temperature as a proportional measure of energy. When two systems are in thermal equilibrium with a third, they are in equilibrium with each other. This principle might seem straightforward but serves as the foundational underpinning for defining temperature scales. By recognizing the transitive property of thermal equilibrium, it gives clarity to how we measure and understand temperature in relation to heat transfer. The ability to define temperature consistently is invaluable in both scientific research and practical applications, from meteorology to engine efficiency.
First Law of Thermodynamics
The First Law of Thermodynamics, also known as the law of energy conservation, states that energy cannot be created or destroyed, only transformed. This principle is at the heart of energy management in all systems, emphasizing that the total energy in an isolated system remains constant.
Energy Conservation
Focusing on energy conservation, this aspect highlights the transfer of energy between systems or its transformation from one form to another. For example, when a car engine converts chemical energy from fuel into mechanical energy to move the vehicle, the total amount of energy remains unchanged, merely shifting form. This reliability in energy conservation not only supports the world's physical laws but also provides a framework for engineering advancements, ensuring efficient designs and processes. Thus, its pivotal role makes it a celebrated principle in discussions about energy efficiency and environmental sustainability.
Work and Heat
Work and heat are the two ways through which energy is transferred in thermodynamic systems. Work is defined as energy transfer resulting from mechanical forces, while heat is the transfer of thermal energy due to a temperature difference. Both these forms of energy transfer play a critical role in thermodynamic processes.
The unique feature of work and heat lies in their methods of energy transfer. While work can be more easily quantified and controlled, heat transfer often involves a range of variables, such as material properties and environmental conditions. Their interplay is crucial in developing technologies like refrigeration systems and heat engines, making them significant topics in practical thermodynamic applications, particularly in minimizing energy loss and increasing efficiency.
Second Law of Thermodynamics
The Second Law of Thermodynamics introduces the concept of entropy, asserting that in any natural process, the total entropy of a closed system can never decrease over time. Entropy provides a measure of disorder or randomness, reflecting the inevitable dispersal of energy.
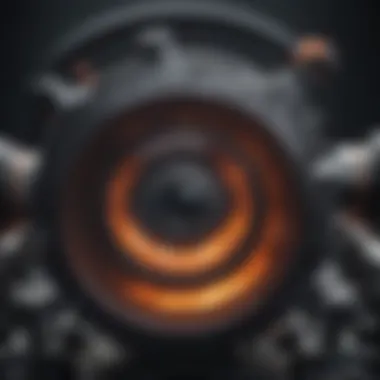
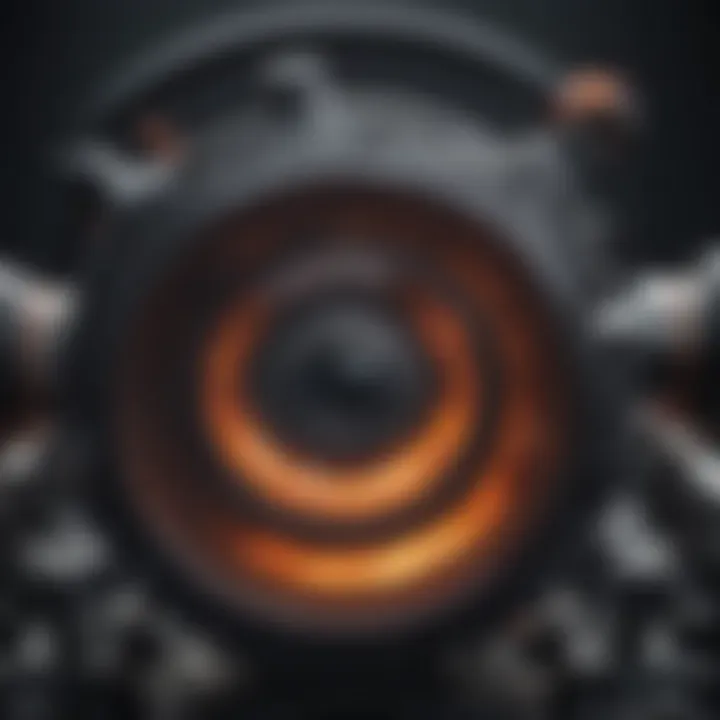
Entropy Increase
The increase of entropy is a vital aspect that underpins various processes in physics and engineering. It posits that systems tend to evolve towards thermodynamic equilibrium, which corresponds to the state of maximum entropy. This insight is paramount in fields addressing energy dissipation and the efficiency of processes, such as in heat engines that convert heat to work. The uniqueness of entropy increase is its capacity to explain why certain processes are irreversible and its implications for sustainability practices, prompting innovations that aim to reduce energy wastage.
Heat Engines
Heat engines are practical applications of the second law, designed to convert heat energy into mechanical work. They illustrate the efficiency limits imposed by entropy, as part of their energy will always be lost as waste heat. Understanding the operation of heat engines, such as the Carnot engine, reveals important implications on efficiency and performance. Their efficiency, typically less than 100%, highlights the importance of optimizing designs and operating conditions, paralleling contemporary discussions on energy use and environmental responsibility.
Third Law of Thermodynamics
The Third Law states that as a system approaches absolute zero, the entropy of a perfect crystal approaches a constant minimum. This law suggests that it is impossible to reach absolute zero in a finite number of steps, a principle that has far-reaching implications in the study of thermodynamics and cryogenics.
Absolute Zero
Absolute zero, defined as zero Kelvin or -273.15 degrees Celsius, represents a state where molecular motion ceases. This concept is pivotal as it sets a limit on how low temperatures can theoretically go. The achievement of near-absolute-zero conditions has enabled advancements in various fields, particularly in quantum mechanics and superconductivity, making it a headliner in discussions about the limits of thermodynamic principles and materials science.
Implications for Entropy
The implications for entropy at absolute zero suggest that the disorder of a system is minimized, approaching perfect order. However, reaching this state is impossible, illustrating the law’s philosophical and practical constraints. This concept has prompted dialogue surrounding thermodynamic laws and performances at lower temperatures, inviting exploration into unconventional states of matter and potential applications in future technologies.
The laws of thermodynamics not only provide a framework for understanding physical processes but also guide the development of technology and sustainability practices in a rapidly changing world. Each law complements the others to build a coherent narrative that stretches across multiple disciplines, highlighting the interconnectivity of energy processes and their real-world applications. By examining the implications, challenges, and advantages of these laws, we can better appreciate the essential role they play in our scientific understanding and technological advancements.
Applications of Thermodynamics
Thermodynamics isn't just theoretical mumbo jumbo; it's the backbone of countless real-world applications. The importance of thermodynamics spans various fields, influencing everything from cutting-edge engineering to the delicate balance of life itself. With a solid understanding of thermodynamics, professionals can innovate solutions that cater to energy efficiency, environmental sustainability, and biological processes. This section dives into those practical applications, highlighting how they intertwine with the principles and laws we've discussed.
Thermodynamics in Engineering
Engineering melds science with practical application, and thermodynamics plays a pivotal role in this field. Engineers utilize thermodynamic principles to design systems aimed at maximizing efficiency and minimizing waste.
Thermal Systems
Thermal systems are everywhere in these applications. They work by transferring heat energy from one medium to another. A key characteristic here is energy transfer, particularly through conduction, convection, or radiation. In designs like car engines or power plants, these systems optimize the conversion of fuel energy into useful work. The compelling element of thermal systems is their ability to provide significant energy efficiency — a hot topic in today's eco-conscious world.
However, they are not without challenges; for instance, material limits and environmental impacts can be a sticking point. Still, their advantages in converting thermal energy into mechanical work make them an essential feature of modern engineering.
Refrigeration Cycle
The refrigeration cycle showcases thermodynamics in action, chilling everything from your fridge to heavy industrial coolers. This cycle is built around a key characteristic: heat absorption and rejection. By utilizing a refrigerant that evaporates at low temperatures, it effectively harnesses heat from inside a space and expels it outside. This process is highly beneficial as it underpins food storage, air conditioning, and even industrial processes.
However, the unique feature of the refrigeration cycle lies in its delicate balance of pressure and temperature. In implementing these systems, an engineer must be cautious about environmental impacts, especially concerning refrigerants that could harm the ozone layer. Thus, while refrigeration is hugely popular and useful, there are continuous efforts to develop more sustainable alternatives.
Biological Implications
Biology, at first glance, might seem distant from thermodynamics. However, thermodynamic principles are vital for understanding biological processes, connecting the dots between energy usage and life itself.
Metabolism
Metabolism exemplifies how thermodynamics governs biological mechanisms. This intricate web of chemical reactions converts food into energy, allowing organisms to grow, reproduce, and maintain vital functions. A key characteristic of metabolism is its dependence on energy transfer, which occurs through metabolic pathways. Here, it’s not just about food – the efficiency of these energy transformations directly impacts an organism's health and adaptability.
What makes metabolism fascinating is its dynamic nature. It can adapt based on environmental conditions, showing how flexible living systems can be. However, this adaptability also comes with potential disadvantages – metabolic disorders can arise when these processes fail. Thus, while metabolism is a necessity for life, it's a double-edged sword that researchers in both thermodynamics and biology strive to understand better.
Homeostasis
Homeostasis is about maintaining equilibrium within an organism—a biological thermostat of sorts. This discipline showcases how energy balances are essential for survival. One of its key characteristics is the feedback loop, which helps organisms regulate temperature, pH, and other vital variables despite external changes. This principle is a beneficial choice for this article because it underlines the myriad ways thermodynamics creates stability in biological systems.
Unique features of homeostasis include its dependence on thermodynamic principles, such as energy exchanges. It enables complex systems to thrive, albeit with the possible downside of vulnerability to environmental shifts. For instance, drastic changes can disrupt an organism's homeostatic balance, leading to severe consequences. In summary, while homeostasis is crucial for life, it illustrates the delicate dance of thermodynamic processes in living organisms.
Environmental Considerations
As the world faces increasing environmental challenges, the intersection of thermodynamics and environmental science offers a lens to examine sustainable practices and energy usage.
Energy Sources
When it comes to energy sources, thermodynamics lays the foundation for understanding how different options—be it fossil fuels, solar, wind, or nuclear—perform in converting energy. A crucial aspect of energy sources is their efficiency, which often dictates their viability in a sustainable world. Renewables have gained ground because they often boast high efficiency and low environmental impact.
However, each source holds unique features that come with trade-offs. Solar energy, while clean, can be limited by weather and requires extensive land use, while fossil fuels, despite being effective, contribute to global warming. Thus, knowledge of thermodynamics plays a crucial role in energy evaluation and strategic planning.
Climate Change
Talking about environmental considerations, climate change can't be ignored. Thermodynamic principles explain how greenhouse gases trap heat, contributing to global warming. A key characteristic of climate change is the complex feedback loops that exacerbate these effects. This aspect makes it a vital topic in our article, as understanding thermodynamics enables researchers and policymakers to devise solutions.
The unique feature here is how thermodynamics bridges our understanding of energy transformations and environmental impacts. By acknowledging these principles, we can tackle the challenges associated with climate change more effectively. However, the downside includes the difficulty in achieving immediate actions or changes as societal norms may resist. Thus, while climate change is a pressing issue grounded in thermodynamic principles, tackling it will require collective effort and transformation.
Modern Interpretations and Theories
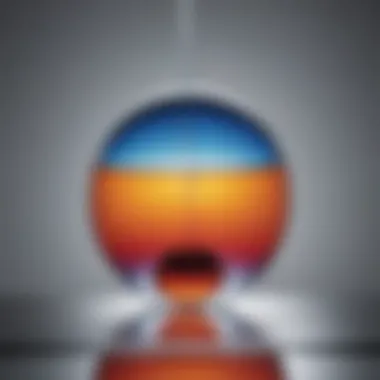
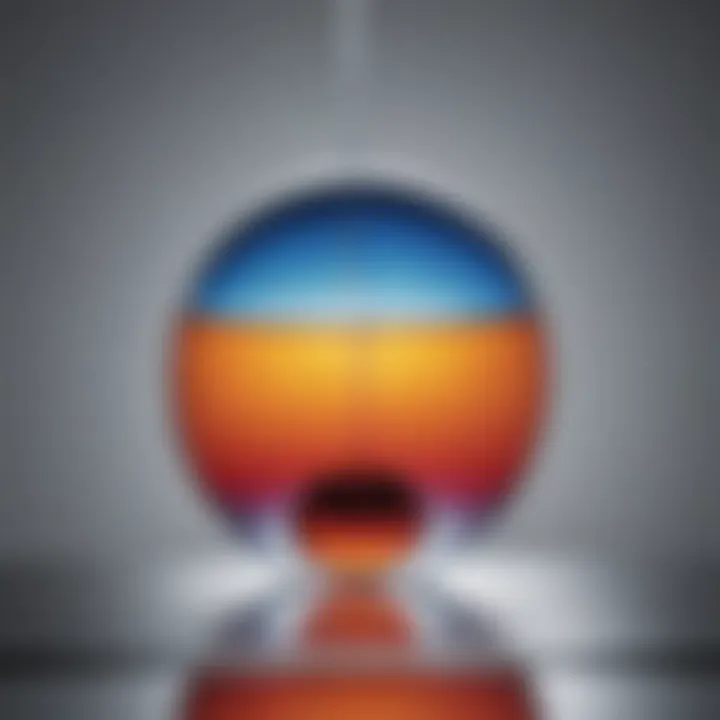
Understanding thermodynamics today requires diving into both modern interpretations and evolving theories. These contemporary perspectives not only enhance our understanding of classic thermodynamic principles but also bridge the gap between theoretical concepts and practical applications. This section aims to illuminate the relevance of statistical mechanics and non-equilibrium thermodynamics as vital components of modern thermodynamic discourse. Through exploring these theories, we gain insight into how they impact various scientific fields, from engineering to physical chemistry, thereby solidifying their significance in the narrative of thermodynamic definitions.
Statistical Mechanics Perspective
Statistical mechanics forms the backbone of our understanding of thermodynamic properties at a molecular level. It connects the macroscopic properties we observe to the microscopic behaviors of individual particles. The brilliance of statistical mechanics is its ability to explain vast systems' behaviors through probabilistic models based on limited observations.
Molecular Interpretations
The molecular interpretation of thermodynamics embraces the idea that all matter consists of tiny particles in constant motion. One key characteristic here is the profound predictive power this perspective has. Notably, it allows for an understanding of thermodynamic behaviors that is grounded not just in empirical observation but in the fundamental laws governing particle interactions. This perspective has emerged as hugely beneficial in the art of explaining phenomena like phase transitions and critical points effectively.
The unique feature of molecular interpretations lies in their ability to provide a microscopic underpinning for thermodynamic laws. It transforms what can often feel abstract into a tangible science, creating an avenue for educational engagement and foundational research. Still, it's worth noting the potential disadvantages – the complexity of calculations can deter those less versed in mathematical methods, sometimes leaving them feeling overwhelmed. However, the clarity it offers those willing to engage with the mathematics makes it an invaluable choice for comprehending thermodynamics at a deeper level.
Thermodynamic Properties
Thermodynamic properties serve as the essential descriptors characterizing various systems. Observables such as pressure, volume, and temperature quantify a system's state and are invaluable for predicting its behavior. These properties are connected through various equations and laws, like the Ideal Gas Law, expanding their relevance across scientific disciplines. Understanding thermodynamic properties enhances our grasp of energy transformations and physical processes.
One notable characteristic is their extensive applicability; they not only find relevance in physics, but also extend into chemistry and engineering realms. This versatility makes them a popular theme in thermodynamics discussions, as they connect diverse scientific paradigms. However, it’s crucial to recognize that the categorization of these properties can sometimes obscure the underlying complexities of real-world systems. The oversimplification may lead to misunderstandings when applied to systems that display non-ideal behavior. Nevertheless, discussing thermodynamic properties often provides a critical foundation for engaging with more complex systems and phenomena.
Non-Equilibrium Thermodynamics
As we move towards non-equilibrium thermodynamics, the narrative shifts to systems that are not in a steady state. This topic captures the essence of dynamic change where processes are ongoing, and equilibrium hasn’t been achieved. Here, the challenges of understanding arise, as these systems often exhibit behaviors that classical thermodynamics struggles to explain.
Non-equilibrium thermodynamics serves as a lens through which we can view phenomena such as heat transfer, chemical reactions, and biological processes, where equilibrium assumptions break down. One significant contribution of this theory is its application to various fields. For example, in biological systems, maintaining homeostasis represents a constant struggle against equilibrium, adding dimensions to our understanding of life processes.
In summary, modern interpretations of thermodynamics enrich our comprehension of this fundamental discipline, illustrating how traditional definitions meet contemporary scientific challenges. From microscopic behaviors derived from statistical mechanics to the dynamic complexities in non-equilibrium systems, each perspective broadens the scope of thermodynamic applications. Overall, these modern theories help constitute a more holistic view, making it clear that thermodynamics remains an evolving field ripe for exploration.
Challenges and Controversies in Thermodynamics
Thermodynamics is not just a collection of principles; it's a living part of various scientific conversations that often stirs up debates and controversies. Understanding these challenges is crucial for anyone delving into the field, as it keeps one grounded in the realities of applying thermodynamic concepts beyond the classroom. The wrinkles in understanding can lead to creative insights and innovative solutions that advance our grasp of the universe.
One of the central topics of contention in thermodynamics revolves around the interpretation of entropy. This concept, while fundamental, carries nuanced implications that scientists and scholars still grappled with. The debate about what entropy truly represents goes beyond mere thermal measurements; it stretches into ideas of disorder, information theory, and even the directional flow of time itself. Various interpretations have emerged, complicating acceptance across different scientific disciplines.
Interpretation of Entropy
Entropy is often thought of as a measure of randomness or disorder in a system. However, its interpretation is far from straightforward. Some argue that entropy merely quantifies the number of microscopic configurations that correspond to a thermodynamic state. Others, like physicists Leonard Susskind, see it as a fundamental expression of our ignorance about a system's precise state.
This suggests that high entropy states could also indicate a lack of information rather than chaotic disorganization. Such views challenge traditional perspectives held in the realms of both thermal physics and statistical mechanics.
- Qualitative Interpretations:
- Entropy as chaos: A familiar viewpoint where high entropy implies disorder that tends to increase.
- Entropy as information: Where entropy reflects a lack of knowledge about a system's precise configuration.
This confusion leads to difficulties in applying entropy across fields, from cosmology to quantum mechanics, potentially muddling effective communication among scientists in diverse specializations. Understanding entropy will not only sharpen thermodynamics knowledge but also enhance interdisciplinary dialogues.
Thermodynamics of Irreversible Processes
Another significant challenge comes from exploring irreversible processes. Thermodynamics traditionally operates within the boundaries of reversibility of certain processes, particularly in idealized settings. However, many natural processes inherently tread on irreversible pathways—think of the melting of ice on a warm day or the combustion of hydrocarbons in our vehicles.
The challenge here lies in reconciling these real-world phenomena with the laws of thermodynamics. The second law posits that the total entropy of an isolated system can never decrease, leading to the idea of irreversibility being closely tied to entropy production.
- Key Points on Irreversible Processes:
- Implications of Research:
- These processes often involve energy dispersal or consumption, impacting efficiency in natural and engineered systems.
- The study of irreversible thermodynamics also intertwines with areas like heat engines and chemical reactions, demanding a more extensive understanding of energy transfer.
- Better design of thermal systems can be achieved from insights in irreversible thermodynamics.
- It can lead to innovations that help us approach more efficient energy systems.
Understanding the challenges related to entropy and irreversible processes pushes researchers to rethink core thermodynamic concepts, which in turn can inspire solutions to real-world problems.
By acknowledging these challenges and controversies, we find a way to not only deepen our knowledge but also encourage a more robust and dynamic approach to challenging the status quo in thermodynamics. The interpretative debates surrounding entropy and the complexities of irreversible processes serve as a crucial lens through which upcoming advancements can be viewed, keeping thermodynamics relevant in our ever-evolving technological landscape.
Closure
Examining the field of thermodynamics, this article serves to highlight its foundational significance across diverse areas of study, from mechanical engineering to environmental science. The importance of the conclusion cannot be understated. It encapsulates the essence of the discourse and reflects on the overarching themes explored throughout the text. By revisiting key concepts, it allows readers to cement their understanding of essential principles that govern this dynamic science.
Summary of Key Points
In our exploration of thermodynamics, several critical points emerged:
- Thermodynamic Fundamentals: The cornerstone concepts, such as systems and surroundings—be they closed, open, or isolated—lay the groundwork for understanding more complex interactions.
- Laws of Thermodynamics: Each law serves a distinct purpose, from energy conservation to the inevitable increase of entropy, reflecting the natural progression toward disorder.
- Applications Across Disciplines: Thermodynamics finds its relevance in varied fields like biology and engineering. This underscores its universal nature.
- Emerging Theories: Modern views, such as statistical mechanics, enrich our comprehension of thermodynamic phenomena.
- Challenge of Measurements: Interpretations of entropy and analysis of irreversible processes invite debate and further inquiry.
By compiling these points, this article ensures that readers walk away equipped with a robust understanding of thermodynamic definitions and their implications.
Future Directions in Thermodynamic Research
Looking ahead, several promising research avenues await exploration within the field of thermodynamics. Researchers can delve into areas such as:
- Quantum Thermodynamics: Investigating the principles of thermodynamics at quantum levels holds potential for groundbreaking discoveries.
- Non-Equilibrium Systems: Understanding how systems behave outside of equilibrium can lead to greater insights in both theoretical and applied contexts.
- Interdisciplinary Applications: The intersection of thermodynamics with nanotechnology and materials science presents opportunities for innovation.
- Environmental Sustainability: With the growing emphasis on energy efficiency, research aimed at optimizing thermal processes could remain essential.
"The relentless pursuit of knowledge will always lead to deeper understanding in the science of thermodynamics, influencing both the present and future of many disciplines."
In sum, this article not only highlights critical elements of thermodynamics but also paves the way for ongoing dialogue and research in an ever-evolving field.