Exploring Neutrino Light and Its Cosmic Implications
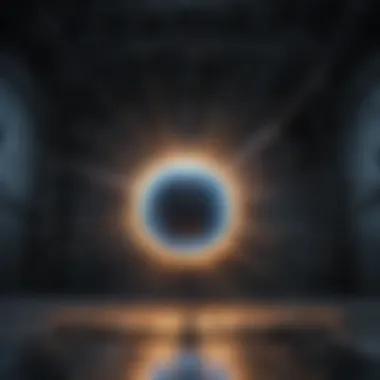
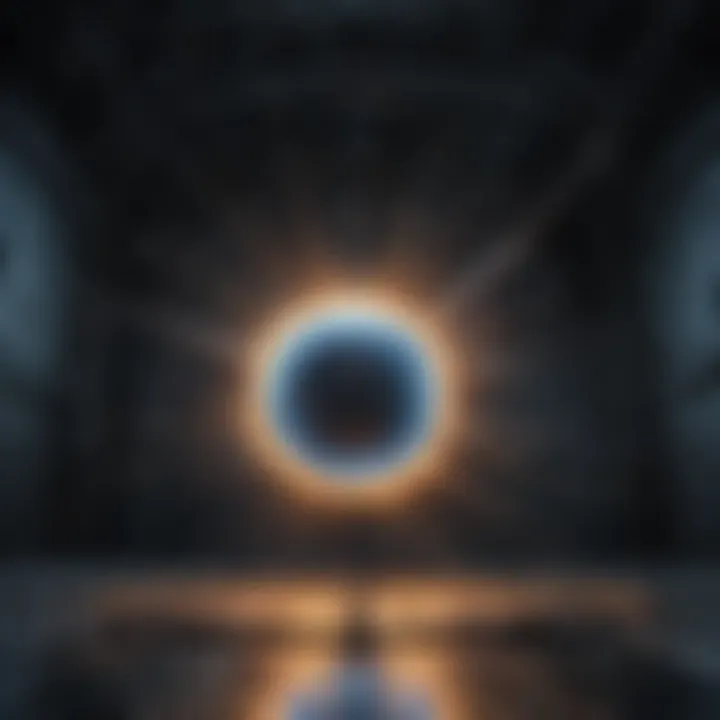
Intro
In the vast expanse of our universe, the role of neutrinos often goes unnoticed. These elusive particles, which carry little to no mass and interact very weakly with matter, constitute an essential aspect of the cosmic fabric. Though practically invisible to our everyday observations, neutrinos are formidable messengers, illuminating the hidden processes that take place in stars and supernovae. As we delve into this realm of neutrino light, we aren't just exploring tiny particles; we’re unraveling some of the most profound mysteries of the cosmos.
To truly grasp the significance of neutrino light, one must first appreciate the fundamental nature of neutrinos themselves. They share a stage with other fundamental particles but inhabit a unique niche in the particle physics landscape. Researchers are continually discovering why these particles are pivotal for our understanding of both the subatomic world and the vast cosmos surrounding us. This article aims to break down those complexities and foster a clearer understanding of neutrino light.
For students, researchers, educators, and professionals alike, this exploration is set to provide a robust overview of the theme, emphasizing recent advancements and theoretical insights that underscore the relevance of neutrinos in modern astrophysics.
The Nature of Neutrinos
Understanding neutrinos is like flipping through the pages of a cosmic mystery novel. Neutrinos are fundamental particles, as small as they come, that whisper secrets of the universe in a language that seems unintelligible at first. Despite being one of the most abundant particles in the cosmos, they evade detection, slipping through ordinary matter like water through a sieve. This elusive nature makes them a fascinating subject of study, leading to significant inquiries in astrophysics and particle physics.
Neutrinos are crucial for comprehending various astrophysical processes, from the heart of stars to intricate phenomena like supernova explosions. Their interactions, albeit weak, give scientists insights into processes that shaped the universe. The discussion around neutrinos is not just academic; it pushes the boundaries of our understanding of matter, energy, and the laws governing them.
Definition and Properties
Neutrinos are lepton particles, meaning they belong to a family that includes electrons and their heavier cousins. Defined by their lack of electric charge and a very small mass, they challenge our perceptions of what particles can be.
- Neutral Charge: Neutrinos do not carry an electric charge, which allows them to interact only through the weak nuclear force and gravity.
- Extremely Light: While once thought to be massless, experiments now show that neutrinos do possess mass, although it’s minuscule compared to that of protons or electrons.
The uniqueness of neutrinos lies in their properties. They come in three flavors—electron, muon, and tau—each associated with a partner charged lepton. This flavor oscillation is a key point in neutrino physics, as it signifies that neutrinos can change types, rarely seen in other particles.
Types of Neutrinos
When discussing neutrinos, it’s essential to differentiate between their types. This classification leads to a better understanding of their roles in various reactions within the universe. As mentioned, there are three genres of neutrinos correlated with their related charged lepton:
- Electron Neutrinos (νₑ): These are produced in processes like nuclear fusion in the sun.
- Muon Neutrinos (νₓ): Generated in the interactions of muons, these neutrinos are of key interest in high-energy physics experiments.
- Tau Neutrinos (νₜ): Although less frequently observed, tau neutrinos emerge from processes involving tau particles.
Each type plays a significant role in the suite of interactions that occur throughout the universe, helping build the foundation for our understanding of the particle world.
Mass and Charge Discussions
The nuances of neutrinos' mass and their charge—or lack thereof—initiate a web of discussions in the realm of physics. For a long time, neutrinos were assumed to be massless. However, the discovery of neutrino oscillation revealed that they have a small but finite mass. This factor raises questions about how neutrinos fit into the larger framework of particle physics, particularly in relation to the Standard Model.
- Mass: The precise measurement of neutrino mass is ongoing, with recent estimates suggesting they are much lighter than previously thought.
- Charge: As stated earlier, neutrinos lack electric charge, resulting in their minimal interaction with matter. This feature contributes to their uncanny ability to travel vast distances through space without a hitch.
"Neutrinos are like ghosts, able to glide through the cosmic landscape, almost undetectable yet profoundly influential in the anatomy of the universe."
Neutrino Light: A Conceptual Overview
The study of neutrino light opens an exciting chapter in our quest to grasp the universe's deeper mysteries. Engaging with this concept helps illuminate not just how these elusive particles operate but also the broader implications they carry in our understanding of physics. By dissecting neutrino light, we can delve into the subtleties of particle interactions and their representation of the overarching cosmic framework.
One of the key elements of neutrino light is its role in bridging various fields of study; from cosmology to particle physics. The interplay between these fields suggests that understanding neutrinos could unlock new pathways in our scientific inquiry. Moreover, recognizing the implications of neutrino light encourages further exploration of profound questions about the nature of matter and energy.
As we embark on this topic, it's vital to note the benefits neutrino light can provide. For researchers and students alike, engaging with this phenomenon fosters a comprehensive understanding of not only how neutrinos work but also the techniques and technologies employed to study them. Additionally, appreciating the significance of neutrino light may unveil pathways toward new scientific discoveries and theoretical advancements.
In this section, we will break down the concept of neutrino light, starting from its definition to a detailed analysis of its physical principles, drawing parallels with electromagnetic radiation. The exploration will set the stage for appreciating how neutrinos symbolize one of the many layers our universe holds, waiting to be uncovered.
What is Neutrino Light?
Neutrino light refers to the unique phenomenon of neutrinos being able to affect the electromagnetic field and their subsequent interactions in a way that produces observable effects. Unlike visible light, which relies on photons, neutrino light involves neutrinos, which are neutral and extremely light.
These particles are created during various cosmic events, such as during stellar processes or supernova explosions. Despite their elusive nature, they flood the universe, passing through ordinary matter almost completely unnoticed. This aspect prompts interesting questions about how we can effectively observe and interpret their influence.
In a practical sense, defining neutrino light involves understanding the conditions under which neutrinos can generate detectable signals. This understanding is crucial for developing detection technologies and expanding our theoretical models concerning the subtle workings of the universe.
Physical Principles Behind Neutrino Light
The concept of neutrino light is deeply rooted in quantum mechanics and relativity. Neutrinos interact through the weak nuclear force, a fundamental aspect of particle physics. Their unique properties, such as very low mass and the absence of electrical charge, mean that they can travel great distances without significant interaction with matter.
One significant aspect of neutrino light is related to the idea of neutrino oscillation, where neutrinos change types as they propagate. This phenomenon reinforces the notion that neutrinos possess mass, a revelation that challenged previous paradigms in physics.
A notable principle peeking behind the curtain of neutrino light is the energy-mass relationship described by Einstein’s theory of relativity. As neutrinos acquire energy through various cosmic processes, they produce effects that resonate through their interactions, albeit subtly.
Comparison with Electromagnetic Radiation
When drawing comparisons between neutrino light and electromagnetic radiation, several core distinctions emerge. Electromagnetic radiation, which includes visible light, travels through the universe at the speed of light and requires charged particles to generate its waves. In contrast, neutrino light does not depend on charge to propagate; it can move through materials like water and stone with a nearly undisturbed trajectory.
Moreover, while electromagnetic radiation can be detected using standard photographic equipment or sensors, detecting neutrinos requires sophisticated detection methods, often involving deep underground chambers or massive detectors like the IceCube Neutrino Observatory in Antarctica.
In summary, we see that though both neutrino light and electromagnetic radiation play critical roles in the fabric of our universe, they operate under vastly different physical principles and characteristics. The interplay between these two forms of light reveals essential truths about our existence and the makeup of the universe.
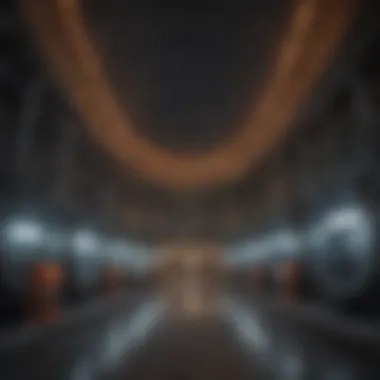
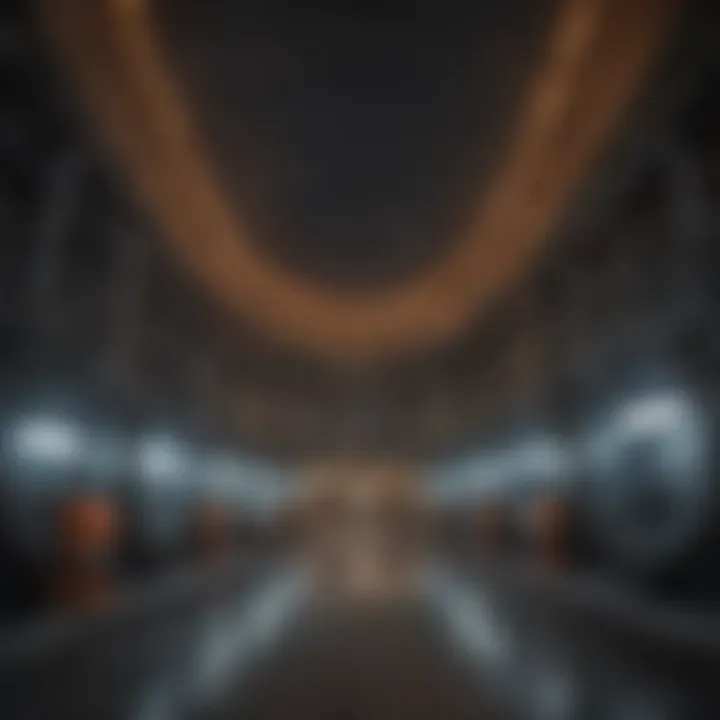
Detection Mechanisms of Neutrinos
Understanding how we detect neutrinos is pivotal to grasping their significance in the universe. Just as a detective pieces together clues to solve a case, scientists use advanced techniques to uncover the secrets that neutrinos hold. Since these elusive particles hardly interact with matter, their detection remains a formidable challenge. However, advancements in detection methods have opened up new avenues for research, enabling a better comprehension of both particle physics and cosmic phenomena.
Historical Development of Detection Techniques
The journey of neutrino detection began back in the 1950s, with the initial work primarily focused on capturing the presence of neutrinos produced by the sun. One of the earliest successful experiments was conducted by Clyde Cowan and Frederick Reines in 1956. They used a large tank of water and measured the resulting interactions after a neutrino collided with a proton, producing a detectable signal. This marked a groundbreaking achievement, earning Reines the Nobel Prize in Physics in 1995.
Since then, various detection techniques have come and gone, evolving to match the scientific and technological demands. The development of particle physics has seen the invention of detectors such as scintillation detectors, which use materials that emit light when energized by particles. Liquid argon detectors, introduced later, allowed for clearer observations due to their ability to capture more interactions, providing a visual representation of neutrino interactions that previously eluded scientists.
Current Detection Methods
Today, several sophisticated methods exist for detecting neutrinos. Each has its unique advantages and focuses on different aspects of neutrino interactions:
- IceCube Neutrino Observatory: Located at the South Pole, this gigantic array of optical sensors detects high-energy neutrinos originating from cosmic sources. By observing Cherenkov radiation caused by particles interacting with the ice, IceCube has opened new frontiers in astrophysics.
- Super-Kamiokande: A renowned water Cherenkov detector in Japan, Super-Kamiokande is notable for its ability to measure atmospheric neutrinos. Its advanced design allows it to observe the faint flashes of light resulting from neutrino interactions in a vast pool of ultra-pure water.
- SNO (Sudbury Neutrino Observatory): Located in Canada, this facility was pivotal in confirming the phenomenon of neutrino oscillation. With its use of heavy water, SNO could distinguish different types of neutrinos, greatly contributing to our understanding of their nature and behavior.
These methods illustrate just how far we have come since the early days of neutrio detection. They not only enhance our knowledge of particle physics but also unveil the underlying processes in astrophysical events, such as supernova explosions and cosmic ray interactions.
Challenges in Detection
Despite the advancements, several challenges persist in the realm of neutrino detection. The primary hurdles include:
- Low Interaction Rates: Neutrinos are famously shy particles. They can pass through light-years of lead without barely grazing a single atom. This makes it exceedingly difficult to detect them unless large volumes of detection material are used.
- Background Noise: In any detector setup, background radiation can create noise that complicates the interpretation of results. Distinguishing genuine signals from these background events is crucial for ensuring the accuracy of the findings.
- Cost and Scalability: The construction and maintenance of large-scale detectors demand significant financial resources. Not all institutions are able to invest in such expansive projects, which can limit the diversity of research facilities across the globe.
"Neutrinos are like whispers from the cosmos; they carry stories from distant stars, yet remain nearly impossible to catch."
Understanding detection mechanisms is not just about measuring these particles—it’s about unraveling the metaphysics of our universe. The quest to understand neutrinos charms scientists and enthusiasts alike, paving the way for future discoveries.
The Role of Neutrinos in Astrophysics
The study of neutrinos is nothing short of pivotal in the realm of astrophysics. These elusive particles, often described as ghostly, provide insights that fundamentally change our understanding of cosmic processes. Neutrinos have a unique ability to traverse vast distances without being absorbed or scattered, which allows them to carry crucial information from their origins all the way to Earth. This quality makes them indispensable in addressing key questions regarding stellar phenomena, structure, and the fundamental aspects of the universe.
Neutrinos and Stellar Evolution
Stellar evolution is the process through which stars form, live, and eventually die. Neutrinos play a significant role throughout different stages of a star's lifecycle. During the nuclear fusion process at a star's core, particularly in stars similar to our Sun, high-energy neutrinos are produced. These particles emerge as byproducts of the fusion of hydrogen into helium and carry away energy from the core.
Neutrinos act as messengers from the heart of a star, helping us to observe and understand the few processes we can’t directly witness.
In massive stars, when hydrogen is depleted, they evolve into heavier elements. As they undergo fusion, like when turning helium into carbon, neutrinos are generated again. The precise measurements of these neutrino emissions can help astronomers grasp the rates of fusion reactions, thus shedding light on the stars’ life cycles.
Supernova Explosions
Supernovae, the powerful explosions that mark the death of certain stars, are another critical area where neutrinos hold great significance. When a massive star reaches the end of its life and undergoes core collapse, it releases an immense burst of energy. During this cataclysmic event, neutrinos are produced in staggering numbers. In fact, studies indicate that during a supernova, neutrinos can outnumber light photons by a factor of nearly one billion.
The study of neutrinos from supernovae is vital, as they provide valuable data regarding the explosion mechanisms and subsequent nucleosynthesis—the formation of new elements in the universe. These observations can also lend insight into the dynamics of the collapsing core and the processes behind the formation of neutron stars. Important historical detections, such as the neutrinos from Supernova 1987A, have cemented this connection, unveiling astonishing details about such monumental events.
Cosmic Ray Interactions
Neutrinos are also essential in understanding cosmic rays, highly energetic particles that originate from various cosmic sources permeating the universe. When these cosmic rays interact with other matter, particularly interstellar gas or radiation from high-energy sources, they can take part in processes that produce neutrinos. For instance, when cosmic rays strike a proton, the interaction can create pions, which further decays into neutrinos.
The study of neutrinos from cosmic rays allows scientists to trace back to their origins and understand more about the energetic processes in places like active galactic nuclei or supernova remnants. This not only aids in mapping cosmic sources but also provides crucial information on fundamental astrophysical processes, linking high-energy physics to cosmological phenomena.
In summary, the role of neutrinos in astrophysics is multi-faceted and deep. Significantly, they connect various aspects of the universe—from the birth and evolution of stars, the explosive events of supernovae, to the mysteriously energetic cosmic rays. Each interaction and emission offers a unique window into the workings and secrets of the universe, making the study of neutrinos indispensable for the continued advancement of astrophysical understanding.
Neutrinos in Particle Physics
The exploration of neutrinos in the realm of particle physics is not just of academic interest; it unveils fundamental principles about the universe itself. Neutrinos are elusive particles, yet their importance cannot be understated in our understanding of the fundamental forces that govern the cosmos. They interact with matter so faintly that they essentially act as messengers from the core of stellar phenomena, providing insights into processes that would otherwise remain hidden from detection.
Neutrino Oscillation Phenomenon
A particularly intriguing aspect of neutrino behavior is the phenomenon known as neutrino oscillation. This is the process through which a neutrino changes its flavor as it travels through space. Essentially, neutrinos come in three types: electron, muon, and tau neutrinos. As these particles race about, they can oscillate between these types, which has profound implications for both theoretical and experimental physics.
To illustrate, consider a beam of muon neutrinos generated in a laboratory setting. As they travel, some of these muon neutrinos might transform into electron neutrinos before they are detected. This unexpected behavior challenges traditional views on particle conservation and calls for reevaluation of our understanding of mass.
Much of this oscillation can be traced back to the mass differences between the neutrino types. Although neutrinos were once thought to have no mass at all, recent findings indicate they carry a small but significant mass. These tiny masses contribute to the oscillation by creating a complex quantum interplay known as lepton mixing. The precise mechanisms of these oscillations remain a hot topic in research, offering numerous avenues for exploration.
Implications for the Standard Model
When we examine the implications of neutrinos and their oscillation for the Standard Model of particle physics, we find that they pose challenging questions. The Standard Model traditionally has a firm footing concerning leptons and quarks, yet neutrinos, with their unexpected mass and behavior, leave room for doubt. The introduction of mass for neutrinos necessitates modifications to the existing framework as it was originally conceived; it was built on the premise that all particles involved are massless.
This revelation begs the question: how should we adapt our understanding of the forces that govern particle interactions? The inclusion of neutrinos into the theoretical fold requires new physics that can accommodate their dynamic properties without contradicting everything we know so far.
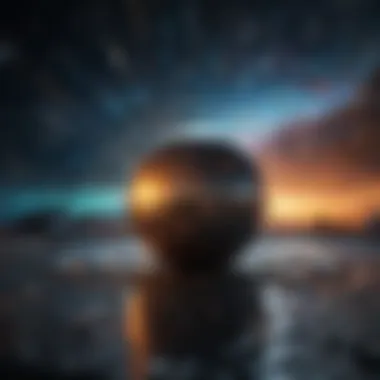
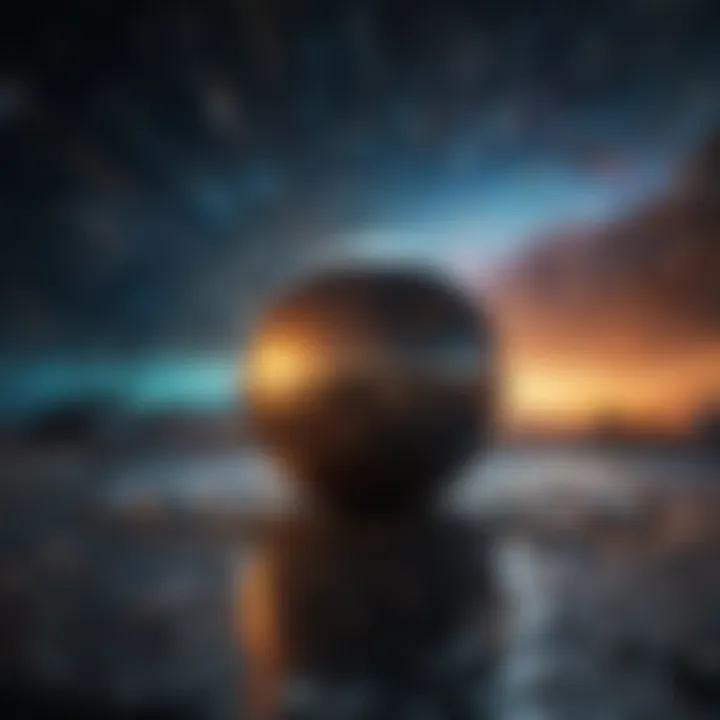
- Key considerations include:
- Mass Generation: What mechanisms allow neutrinos to acquire mass?
- Unification: Can we develop a model that unifies neutrinos with other particles across the Standard Model?
- Experimental Verification: How do current experiments confirm or challenge proposed theoretical frameworks?
Beyond the Standard Model: What Lies Ahead?
Peering into the future, the landscape of neutrino research hints at exciting possibilities that could extend beyond the standard model. These breakthroughs may pave the way for more profound questions about the universe.
For instance, theories such as supersymmetry and various grand unified theories (GUTs) speculate on the nature of neutrinos within larger frameworks. Researchers are exploring what gaps in our current knowledge these particles can fill, pondering questions like:
- What role do neutrinos play in dark matter theories?
- Could neutrinoless double beta decay lead to insights into why the universe contains more matter than antimatter?
The journey into the unknown is fraught with challenges, of course. Technological limits still hinder our ability to conduct certain experiments that could provide clearer answers. However, the continued collaboration among physicists globally is promising efficient paths toward unveiling the mysteries. As experiments evolve and technology advances, the narrative surrounding neutrinos must be rewritten, one oscillation at a time.
"Neutrinos are the whispers of the universe, subtle yet profound, shaping our understanding without ever putting themselves in the spotlight."
Understanding neutrinos and their role in particle physics is critical for advancing our grasp of the fundamental forces of nature. As research progresses, we stand on the brink of breakthroughs that could transform our comprehension of not just particles, but of the universe itself.
Neutrino Experiments and Observatories
The field of neutrino research has evolved significantly, particularly in the context of experiments and observatories dedicated to the study of these elusive particles. Understanding neutrinos isn't just about their theoretical properties; it’s deeply rooted in how scientists actually observe and measure them. Experiments provide crucial insights that inform our comprehension of the universe, and observatories help in gathering data from these particles that are constantly passing through us, often unnoticed.
Neutrino experiments help reveal the fundamental nature of these particles, examining not only their existence but also their interactions with matter and within other domains of physics. By gathering concrete evidence from experiments, scientists are equipped to tackle profound questions about the fabric of the universe, such as the balance of matter and antimatter, and potential pathways to new physics beyond the Standard Model.
In addition, the collaborative nature of these experiments fosters international partnerships, allowing diverse contributions of ideas and resources. This collaboration leads to enhanced productivity and innovations in detection technologies, crucial for pushing the envelope in neutrino science.
Key Experiments in Neutrino Research
Some key milestones in neutrino research have been pivotal in transitioning from theoretical speculation to accepted scientific knowledge. For instance, the Homestake Experiment, which began in the late 1960s, was the first significant detection of solar neutrinos. Its results prompted further inquiries into what could account for the observed deficit in the expected number of neutrinos from the Sun, thus opening up avenues for studying neutrino oscillation—wherein neutrinos change types as they travel.
Another landmark experiment is the Super-Kamiokande in Japan. This facility has not only confirmed the existence of neutrino oscillation but also provided evidence that neutrinos have mass, which was a groundbreaking revelation. Similarly, experiments like NOvA and DUNE represent the next steps in our capabilities, aiming to delve deeper into the asymmetries between neutrinos and antineutrinos.
- Homestake Experiment: First to detect solar neutrinos, highlighting disparity in expected vs. actual observations.
- Super-Kamiokande: Confirmed neutrino oscillation and established neutrino mass.
- NOvA and DUNE: Next-generation experiments focused on exploring fundamental properties of neutrinos.
Neutrino Observatories Around the World
Across the globe, numerous observatories serve as crucial hubs for neutrino research. Each facility possesses unique characteristics tailored to the specific type of neutrino observations. For example, the IceCube Neutrino Observatory in Antarctica is an innovative facility nested beneath the ice, which uses over 5,000 sensors to detect neutrinos emerging from astrophysical sources, such as supernovae and gamma-ray bursts. The design of IceCube allows it to capture high-energy neutrinos originating from deep space.
Gran Sasso National Laboratory in Italy is another central player in the field. It comprises a variety of experiments aimed at studying neutrinos produced from nuclear reactors, atmospheric interactions, and solar emissions. Such observatories are placed strategically to minimize background noise from other cosmic rays, delivering cleaner data for analysis.
Collaborative Efforts in Research
Collaboration plays a vital role in enhancing neutrino research. Partnerships among various countries and institutions broaden the scope of testing hypotheses, sharing technologies, and achieving breakthroughs. For instance, the Joint Institute for Nuclear Research, alongside facilities like Fermilab in the United States and CERN in Europe, engage in sizable collaborative endeavors such as the T2K experiment, which studies neutrino oscillation with a beam directed from Japan to Canada.
Efforts such as these underscore a collaborative spirit in scientific discovery, allowing for shared resources and pooling intellectual capital.
"Alone we can do so little; together we can do so much." — Helen Keller
Together, these elements of experiments, observatories, and collaborative research underscore the dynamic and international effort required to unravel the complexities of neutrinos. Such studies not only illuminate the inherent traits of these fundamental particles but also illuminate their consequential roles in the broader cosmic narrative.
Future Directions in Neutrino Research
Neutrino research is like staring into a vast ocean, with countless depths yet to explore. Understanding the future directions in this field holds great promise, offering not just advancements in detection technologies but also profound insights into the very structure of our universe. These future pathways are pivotal, as they inform not just scientists, but also students, researchers, and enthusiasts about what might lie ahead in our cosmic journey.
Predicted Advances in Detection Technology
As researchers strive to catch glimpses of elusive neutrinos, technology is stepping up to the plate with innovative solutions.
- Improved sensors and detectors are on the horizon, potentially allowing for greater sensitivity to neutrino signals. For instance, advances in semiconductor technology could lead to detectors that take advantage of new materials, increasing efficiency and reducing noise.
- Integration of machine learning and artificial intelligence can greatly enhance data analysis. These tools can help sift through the massive amounts of information generated by neutrino detection, pinpointing significant patterns that might otherwise go unnoticed.
- Innovative designs for undersea or underground detectors might expand our horizons. By situating these devices in extreme environments, scientists could mitigate background noise and focus on the pure signal generated by neutrinos.
The focus on developing compact and portable systems can also be beneficial, making neutrino detection more accessible globally, even to smaller research institutions.
Potential Discoveries in Astrophysics
With advanced detection techniques, the potential to uncover new astrophysical insights becomes clearer.
- Probing the early universe: Neutrinos could provide a unique window into the conditions of the universe shortly after the Big Bang. Understanding their role in that formative period could reshape cosmological models and enhance our grasp of the universe’s origins.
- Investigating cosmic phenomena: Higher sensitivity detectors might soon observe neutrinos from distant supernovae or gamma-ray bursts. Such discoveries could shed light on stellar processes and the lifecycle of stars, revealing links between neutrinos and the fundamental shifts in cosmic activity.
- Hidden physics: Neutrinos might play a role in phenomena that are not yet fully understood. For example, the characteristic blasts from neutron stars may yield insights into gravitational waves and the violent forces at play in the cosmos.
Long-term Implications for Physics
As the field of neutrino research expands, its implications for physics grow multifaceted.
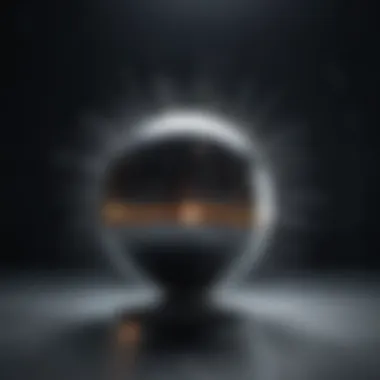
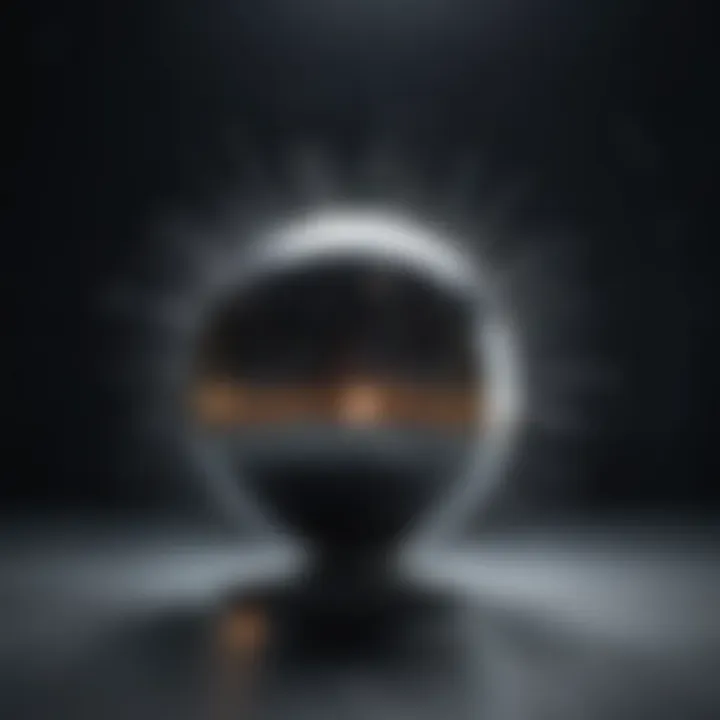
- Refining the Standard Model: The more we learn about neutrinos, the more we may rethink existing theories. Neutrino oscillations challenge current frameworks, suggesting that there might be physics beyond what we've established so far.
- Quantum mechanics and gravity: The intersections of neutrino behavior with quantum mechanics could eventually lead to breakthroughs in understanding gravity at quantum scales. This can have vast consequences for theoretical physics, potentially leading to a more unified understanding of forces.
- Philosophical implications: The exploration of neutrinos also opens the door to philosophical questions about the nature of reality and existence. Understanding these particles might change our perception of the cosmos as a whole.
"Neutrinos are like ghostly messengers from the stars, with the power to change our understanding of the universe's very core."
In summary, the future directions in neutrino research are brimming with potential to redefine our comprehension of both astrophysics and fundamental physics. As detection methods become more advanced, we stand at the threshold of significant discoveries that may illuminate the dark corners of our universe.
Neutrino Light in Cosmology
Neutrinos aren’t just elementary particles whizzing around in the universe; they play a significant role in cosmology. Their lightweight nature allows them to pass through matter almost undetected, providing a unique insight into the processes that shaped our universe. The study of neutrino light helps in shedding light on various cosmic phenomena, helping us piece together the historical puzzle of the universe’s formation and evolution.
The Cosmic Neutrino Background
One of the most pivotal aspects of neutrinos in cosmology is their contribution to the Cosmic Neutrino Background (CNB). This foundational component of the universe is akin to the Cosmic Microwave Background radiation, but instead of photons, it's a sea of neutrinos permeating space. Created just seconds after the Big Bang, these neutrinos provide a glimpse into the conditions of the early universe.
- The essence of the CNB:
- The CNB is believed to contain around ions of neutrinos that were released during the cooling phase of the universe.
- These neutrinos travel through space virtually untouched by their surroundings, their existence forming a sort of ethereal background noise that can affect the behavior of other particles over cosmic scales.
Researchers harness tiny detectors to analyze the faint signals from the CNB. Discovering the properties of these neutrinos allows scientists to infer information about the early universe's temperature, density, and overall structure—key clues locked away in the cosmic expanse.
Inflation and Neutrino Contributions
The concept of inflation refers to a rapid expansion of space in the early universe, which could explain many observations from the cosmos today. But, how do neutrinos fit into the puzzle of inflation? Well, neutrinos contribute significantly to the understanding of this phenomenon. As spacetime expanded and cooled, neutrinos served as crucial players in shaping the mass-energy composition of the universe.
- Neutrinos’ impact on inflation:
- They influence the dynamics of inflation by contributing to the energy density during the expansion. This density must align with observed cosmological evidence, such as the distribution of galaxies and the uniformity of the Cosmic Microwave Background.
- Furthermore, neutrinos help model the fluctuations in density that resulted in the cosmic structure we see today, providing solid grounds for understanding why galaxies formed as they did.
Challenging Existing Cosmological Models
Neutrinos have the potential to challenge and refine existing cosmological models. Some theories propose that neutrinos might affect the rate of cosmic expansion, thus prompting scientists to re-evaluate models of dark energy. If neutrinos possess mass, even a small amount, they could influence the universe's fate in unexpected ways.
- Key points of challenge:
- The standard model of cosmology might need modifications if new findings suggest that neutrinos play a more active role in cosmic evolution than previously thought.
- Observations regarding neutrino interactions connect directly to dark matter theories, raising questions on how we perceive and calculate cosmic structures.
Thus, the interplay between neutrinos and the expanding cosmos reveals an intricate tapestry at work, shedding light on longstanding mysteries while opening the door to new theories and investigations. Understanding neutrinos is not just about studying particles; it’s about understanding the very fabric of our universe.
The Interconnectedness of Neutrinos and Quantum Mechanics
Understanding the relationship between neutrinos and quantum mechanics is pivotal for grasping the subtleties of our universe. Both areas delve into the fundamental structure of reality, where particles like neutrinos play crucial roles in the dance of quantum interactions. This connection is noteworthy for several reasons:
- Fundamental Particle Insight: Neutrinos are the ghosts of the particle world, widely recognized for their elusive nature. Their behavior under quantum mechanics not only reveals intrinsic properties but also informs us about the fundamental forces shaping our cosmos.
- Emerging Theories: Quantum mechanics is continuously evolving, and insights gained from studying neutrinos can challenge and refine existing theories. By embedding neutrinos within quantum frameworks, researchers explore new territories that stretch the understanding of particle interactions and the forces at play.
- Practical Applications: Beyond theoretical implications, working with neutrinos in quantum contexts opens doors to practical advancements—everything from improved detection technologies to advances in quantum computing.
With these considerations in mind, we now delve deeper into the interconnected layers of neutrino studies within quantum mechanics.
Neutrinos within Quantum Field Theory
Neutrinos fit neatly into the framework of quantum field theory (QFT), which describes how particles interact through fields. In this tableau, neutrinos are viewed as excitations in their respective fields, playing a significant role in weak interactions. Unlike other particles, neutrinos travel great distances, essentially slipping through matter as if it were water.
This unique trait has consequences in QFT:
- Yukawa Coupling: The interaction of neutrinos with other particles occurs via weak forces, mediated by gauge bosons. This coupling helps physicists understand mass generation and decay processes.
- Chirality and Helicity: Neutrinos exhibit distinct chirality states, which influences their interactions and assists in manipulating quantum states as needed in experiments.
Exploring these characteristics through QFT enriches our grasp of neutrino behavior and supports the ongoing quest for a unified understanding of the standard model.
Entanglement and Neutrino States
Entanglement is a major pillar of quantum mechanics, where two particles become intertwined, such that the state of one instantaneously affects the other, regardless of distance. When it comes to neutrinos, this phenomenon presents a fascinating twist.
Here’s what sets them apart:
- Nonlocality: Neutrinos can become entangled during their interactions, leading to implications that transcend classical understanding. This nonlocal character challenges preconceived notions about locality in quantum mechanics.
- Oscillation Ties: The phenomenon of neutrino oscillation, where a neutrino changes its type as it travels, can be viewed as an entanglement between different neutrino states. This forms a bridge between quantum state changes and concrete observations in particle physics.
Memorizing these entangled states encourages a broader query about their implications for quantum systems and their potential applications in future technologies.
Implications for Quantum Computing
The exploration of neutrinos in the context of quantum computing is an emerging frontier that holds substantial promise. When neutrinos are incorporated into quantum computing frameworks, they present several advantages:
- Robustness Against Decoherence: Neutrinos are minimally interactive and appear to resist decoherence strongly, a downfall for traditional quantum systems. This could lead to more stable qubits that are less prone to environmental noise.
- Information Encoding: Given their properties, neutrinos can be utilized to encode information in novel ways, potentially enhancing computational power and processing capabilities.
- Networking of Quantum States: Utilizing neutrinos could pave the way for complex networks of quantum states that communicate seamlessly, fostering dense interconnections that mirror classical computing networks but on a quantum level.
As researchers continue to probe the potential of neutrinos in quantum computing, they may reshape our understanding of computation, providing advances in processing speeds and capabilities yet unimagined.
"The subtle interplay between neutrinos and quantum mechanics hints at a richer tapestry of reality, one that beckons exploration and enlightenment."
This intersection invites an ever-deepening discourse, fulfilling a dynamic role in both theoretical and applied physics. The road ahead looks promising and filled with potential discoveries that could redefine our perception of the quantum realm.