Exploring the Complexities of DNA: A Comprehensive Overview
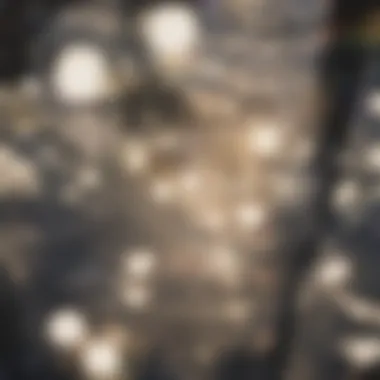
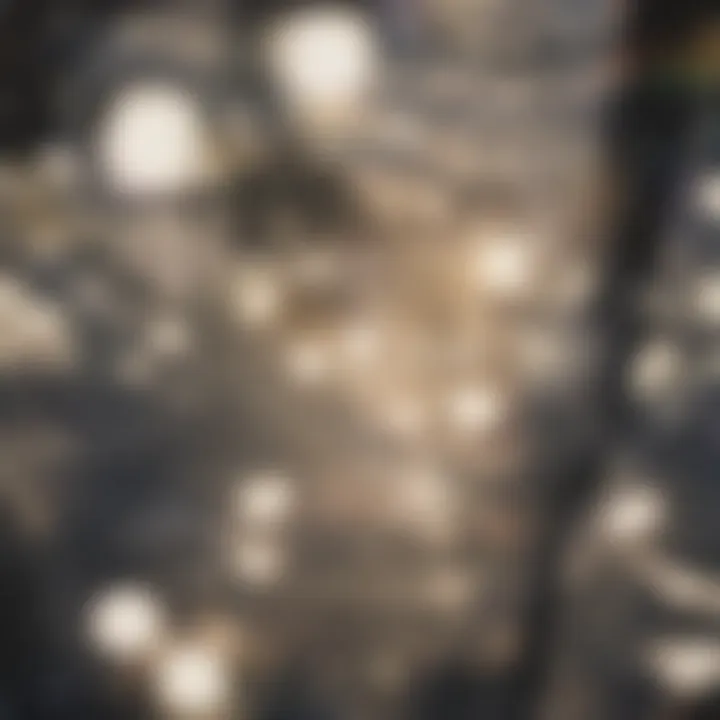
Intro
DNA, or deoxyribonucleic acid, serves as the biomechanical blueprint of life. Its complexity is reflected in its critical role in heredity, cellular function, and biological evolution. The study of DNA encompasses a vast array of scientific fields, from genetics to biotechnology, making it essential for both research and practical applications. This comprehensive overview will guide readers through significant discoveries, fundamental concepts and advancements in DNA research.
The basic structure of DNA looks like a twisted ladder, known as a double helix. This helix comprises nucleotides, which are the building blocks that encode genetic information. Each nucleotide contains a phosphate group, a sugar molecule, and one of four nitrogenous bases: adenine, thymine, cytosine, or guanine. The precise arrangement of these bases determines the genetic code that each organism possesses.
Understanding DNA's complexities offers insights into genetic diseases, evolutionary biology, and innovative biotechnological applications. This article aims to make these intricate topics accessible for students, researchers, educators, and professionals. By demystifying the nuances of DNA, we can better appreciate its significance in the biological sciences and beyond.
Prologue to DNA
Understanding DNA is essential in various fields, from genetics to biotechnology. This knowledge underpins modern biology and medicine, emphasizing how organisms inherit traits and evolve over time. The exploration of DNA provides insight into life's mechanisms, from cellular functions to the interaction of genes with their environment.
As we embark on this detailed overview, it is crucial to recognize why DNA captivates researchers and students alike. It serves as the instruction manual for life, guiding the processes that sustain living organisms. In this section, we will delve into the history of DNA discovery and define what DNA truly is.
The Discovery of DNA
The journey to understanding DNA began in the 19th century when scientific minds started to uncover the building blocks of life. A pivotal moment occurred in 1869 when Friedrich Miescher isolated a substance from the nuclei of white blood cells, which he called "nuclein." This substance, which later became known as DNA, marked the beginning of molecular biology.
In the following decades, researchers like James Watson and Francis Crick took significant steps forward. Their groundbreaking work in the early 1950s established the double helix structure, fundamentally changing our comprehension of genetic information. Watson and Crick built upon the x-ray diffraction images by Rosalind Franklin, leading to the insight that DNA is composed of two intertwined strands.
This discovery had far-reaching implications. It laid the foundation for modern genetics, influencing not only biology but also forensics and medicine.
What is DNA?
DNA, or deoxyribonucleic acid, is the molecule that carries the genetic instructions used in the growth, development, functioning, and reproduction of all known living organisms and many viruses. Structurally, DNA is composed of two long chains of nucleotides twisted into a double helix. Each nucleotide contains a sugar, a phosphate group, and a nitrogenous base. The sequence of these bases encodes the genetic information.
DNA's primary function involves storing and transmitting genetic information. It enables organisms to pass traits from one generation to the next. Every cell in a multicellular organism contains a complete DNA copy, ensuring the continuity and transmission of vital information through processes like replication, transcription, and translation.
In summary, the exploration of DNA and its complexities provides essential knowledge for anyone interested in biology or life sciences. With the understanding of its discovery and its fundamental nature established, we can further investigate its structure and functions.
Structure of DNA
Understanding the Structure of DNA is essential to grasp the complexities of genetic information. DNA, or deoxyribonucleic acid, serves as the blueprint for all living organisms. Its structure not only informs its function but also underpins advancements in various scientific fields, particularly genetics and biotechnology. In this section, we will delve into the double helix formation and explore the key components that make up DNA, including sugars, phosphates, and nitrogenous bases.
Double Helix Formation
The double helix is a fundamental feature of DNA, first described by James Watson and Francis Crick. This structure resembles a twisted ladder, where the sides are composed of alternating sugar and phosphate groups. The rungs of the ladder are formed by pairs of nitrogenous bases, which connect through hydrogen bonds. This unique formation is critical because it allows for the compact storage of genetic information.
Moreover, the anti-parallel nature of the double strands ensures that each strand serves as a template during replication. Without this specific structure, DNA would not provide the stability needed for the inheritance of genetic traits, significantly affecting biological functions.
Components of DNA
Sugars
Sugars are a crucial part of the DNA structure. Each nucleotide comprises a deoxyribose sugar. The importance of the sugar lies in its role in linking the phosphate groups and nitrogenous bases. This connection is vital, as it forms the backbone of the DNA strand. Deoxyribose differs from ribose, found in RNA, by lacking one oxygen atom. This subtle difference gives DNA its stability, making it less reactive than RNA, and contributes to its long-term storage ability.
Phosphates
Phosphates play an equally important role in DNA's structure. Each sugar molecule is linked to a phosphate group, forming a sugar-phosphate backbone. This backbone is essential because it provides structural integrity to the DNA molecule. Phosphates are negatively charged, which helps to stabilize the DNA structure by repelling other negatively charged molecules. The presence of this backbone allows DNA to maintain its helical structure, which is crucial for its functions in replication and transcription.
Nitrogenous Bases
Nitrogenous bases are perhaps the most well-known components of DNA. There are four types of nitrogenous bases: adenine, thymine, cytosine, and guanine. These bases are responsible for encoding genetic information through their specific pairing. Adenine pairs with thymine, while cytosine pairs with guanine. This pairing is critical for the accurate copying of DNA during replication and for the synthesis of RNA during transcription. The varying sequences of these bases determine the specific traits and characteristics of an organism. Understanding nitrogenous bases is therefore indispensable in fields such as genetics, biotechnology, and evolutionary biology.
"The sequence of nitrogenous bases encodes the genetic information that drives all biological processes."
Functions of DNA
The functions of DNA are pivotal in understanding the mechanisms of life. DNA not only carries genetic information, but also directs a multitude of biological functions. Its role is essential in various processes, making it a cornerstone of genetics and biology.
Genetic Information Storage
DNA functions primarily as a storage medium for genetic information. It encodes the instructions necessary for the development, functioning, growth, and reproduction of all living organisms. Each sequence of nucleotides within DNA corresponds to specific traits in an organism. This information is stored in a compact form, allowing for efficient access and replication.
Key points about genetic information storage include:
- Stability: DNA's double helix structure protects it from damage, ensuring that genetic information is preserved over time.
- Replication: When a cell divides, DNA is replicated, allowing genetic information to be passed down to new cells.
- Expression: Specific genes within the DNA can be activated or silenced, leading to cellular differentiation and varied functions among different cell types.
Role in Protein Synthesis
DNA is also fundamental to protein synthesis. This process is critical for cellular activities, as proteins are involved in nearly every function of a cell. The information stored in DNA is translated into proteins through two main processes: transcription and translation. Each step plays an important role in converting genetic codes into functional products.
Transcription Process
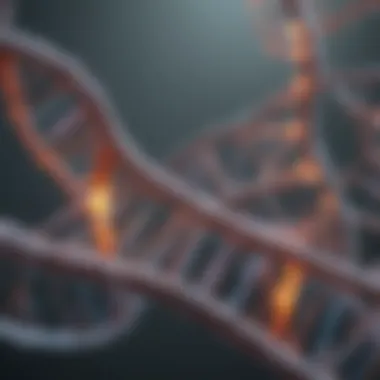
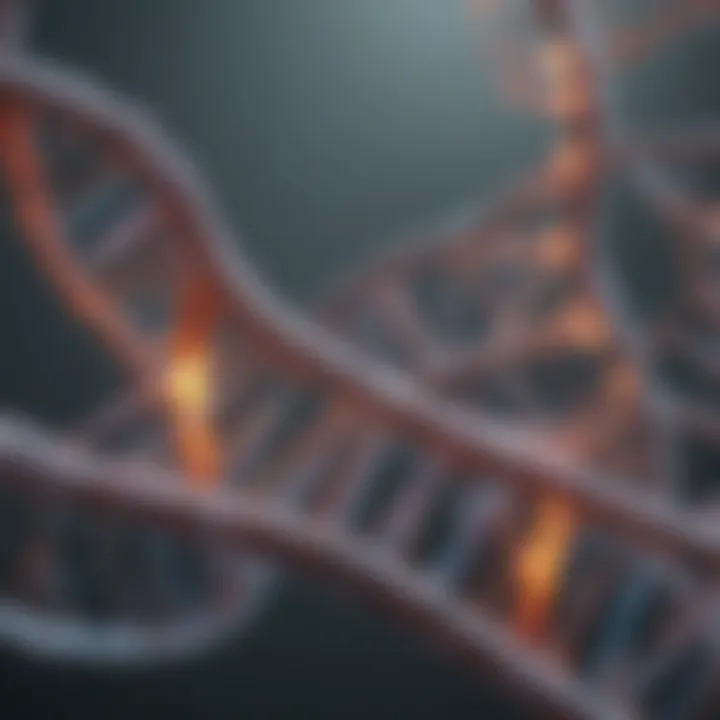
The transcription process involves the conversion of DNA into messenger RNA (mRNA). During transcription,
- A specific segment of DNA is unwound and read by RNA polymerase, which synthesizes a complementary strand of mRNA.
- This process is pivotal because mRNA serves as the template for protein synthesis in the next step.
A key characteristic of transcription:
- It allows cells to respond rapidly to changes in their environment by regulating gene expression.
Benefits of transcription include:
- Efficiency: It can generate multiple mRNA copies from a single gene.
- Regulation: It provides a mechanism to control which proteins are produced, thus influencing cell behavior.
Translation Process
The translation process follows transcription. It occurs in the ribosomes, where mRNA is decoded to build proteins. This involves:
- tRNA (transfer RNA) molecules bringing the correct amino acids that align with the sequence dictated by the mRNA.
- The ribosome facilitates the formation of peptide bonds between amino acids, building a polypeptide chain that will fold into a functional protein.
One important characteristic of translation:
- It allows for diverse protein formation from a relatively small number of amino acids.
Advantages and disadvantages of translation include:
- Advantage: High fidelity in protein synthesis; errors are rare.
- Disadvantage: The process can be influenced by various factors, potentially leading to misfolded proteins if components are dysfunctional.
Overall, the functions of DNA extend beyond mere data storage. They encompass intricate processes essential for life, influencing everything from how organisms grow to how they respond to their environment. Understanding these functions is key to mastering genetics and its implications in various fields.
DNA Replication
DNA replication is a critical process that ensures genetic continuity across generations. Understanding this process sheds light on how biological information is preserved and transmitted. It is central to growth, reproduction, and cellular repair. The fidelity of replication directly impacts genetic stability, making it a vital area of study in molecular biology.
Mechanisms of Replication
The mechanisms of DNA replication involve a series of well-orchestrated steps that ensure accurate duplication of the genetic material. This process begins at specific points in the DNA called origins of replication. Enzymes unwind the double helix structure, allowing access to each strand. As the strands separate, the replication fork is formed. DNA polymerases synthesize the new strands of DNA by adding complementary nucleotides to the existing template strands. This semi-conservative mechanism guarantees that each daughter DNA molecule consists of one original and one newly synthesized strand.
Enzymes Involved
A variety of enzymes play essential roles during DNA replication, each contributing to the success of this intricate process.
Helicase
Helicase is pivotal in unwinding the double-stranded DNA at the replication fork. This enzyme breaks the hydrogen bonds between nitrogenous bases, allowing the two strands to separate. The key characteristic of helicase is its motor activity which requires energy to function effectively. Its activity makes it a beneficial choice for initiating replication. A unique feature of helicase is its ability to move along the DNA strand in both directions, which can speed up the unwinding process. However, the disadvantage lies in the potential for errors during the unwinding phase, which can lead to problems later in replication.
DNA Polymerase
DNA polymerase is fundamental in synthesizing new DNA strands. Its primary role is to add nucleotides to the growing DNA chain. One of the key characteristics of DNA polymerase is its ability to proofread its work. This proofreading function is essential for maintaining fidelity during replication. Its unique feature includes the capacity to extend the DNA strand only from the 3' end, which means new nucleotides are added in a specific direction. A disadvantage of DNA polymerase is its requirement for a primer to initiate synthesis, which adds a layer of complexity to the replication process.
Ligase
Ligase is crucial for joining the Okazaki fragments on the lagging strand. It seals the gaps between the newly synthesized DNA pieces, resulting in a continuous DNA strand. The key characteristic of ligase is its ability to facilitate the formation of phosphodiester bonds. This makes it a beneficial choice in ensuring the integrity of the newly replicated strand. A unique feature of ligase is its activity in utilizing energy from ATP or NAD+ during the ligation process. However, its deficiency in activity could lead to incomplete replication, which can result in significant genetic anomalies.
The accuracy of DNA replication is crucial for maintaining genetic fidelity and preventing mutations that could have significant biological implications.
In summary, DNA replication is an intricate process involving multiple mechanisms and key enzymes. The understanding of these elements contributes significantly to the insights into genetic research and its applications. Each enzyme's distinct roles highlight the importance of coordination and accuracy necessary for successful DNA replication.
DNA Mutations
DNA mutations are fundamental to understanding genetic diversity and evolution. They are changes in the nucleotide sequence of the DNA. These variations can lead to different traits within species or even new species over geological timescales. Understanding mutations is crucial for fields like medicine, genetics, and evolutionary biology as they influence everything from health conditions to the adaptability of populations. There are various types of DNA mutations, each contributing uniquely to genetic variation.
Types of Mutations
Point Mutations
Point mutations are alterations in a single nucleotide base pair in the DNA sequence. They can be classified further into silent, missense, and nonsense mutations. The key characteristic of point mutations is their precision; they change one base without affecting the others. This specificity makes them beneficial for targeted genetic studies or therapies.
The unique feature of point mutations lies in their potential impact on protein coding. For instance, a missense mutation may result in a protein that functions differently than intended, which can lead to diseases. On the other hand, silent mutations do not affect protein function, showing how minor changes can have varied effects.
Insertions and Deletions
Insertions and deletions (often referred to as indels) result when nucleotide bases are added or removed from the DNA sequence. The key characteristic of indels is that they can disrupt the reading frame during protein synthesis, leading to significant changes in amino acid sequences downstream. This can result in nonfunctional proteins or proteins with altered activity, posing risks for causing genetic disorders.
Insertions can introduce new information into a genome, which could be beneficial by adding new functions. Deletions, however, often result in loss of function, leading to more pronounced defects. Such mutations are prominent in various genetic diseases and are crucial in understanding gene function.
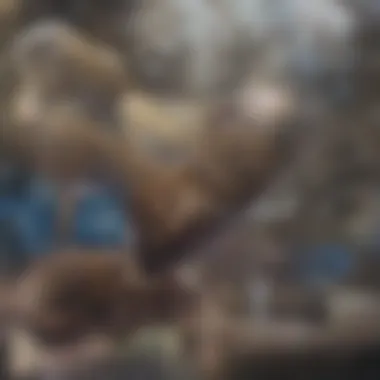
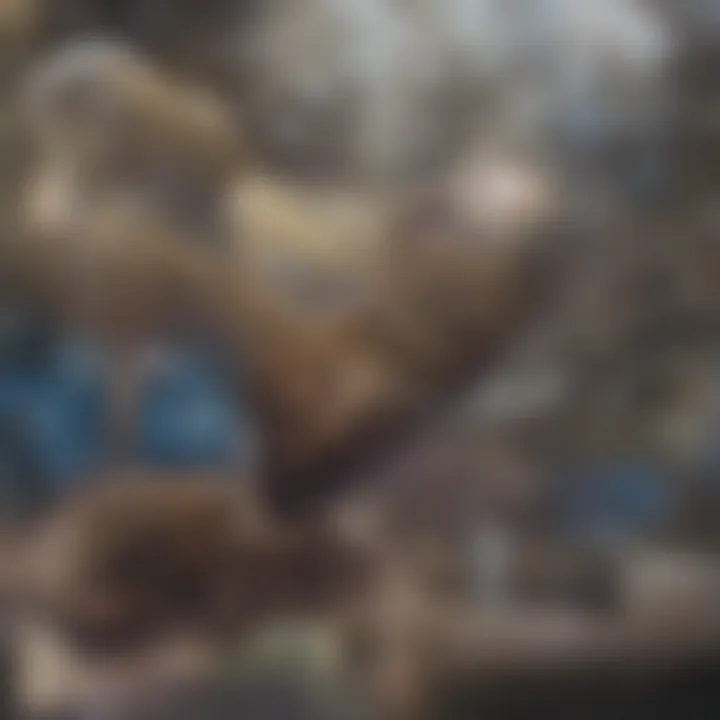
Chromosomal Mutations
Chromosomal mutations involve large-scale changes in the structure of chromosomes. These include duplications, deletions, inversions, or translocations of segments of DNA. The key characteristic here is their complexity and the wide range of impacts they can have. Chromosomal mutations can create significant genetic variability and can be beneficial when they lead to new traits that enhance survival or reproduction.
A unique feature of chromosomal mutations is their potential to affect many genes at once. This can be advantageous in evolution, as it may provide sudden adaptations. However, they can also lead to serious conditions such as cancer or congenital anomalies when the balance of gene dosage is disrupted.
Impacts of Mutations
Mutations can have profound impacts on organisms. They can be beneficial, neutral, or harmful. Beneficial mutations may confer advantages, such as increased resistance to diseases or better adaptation to environmental changes. Neutral mutations usually have no significant effects, serving as genetic variation within populations. Harmful mutations can lead to genetic disorders or increase susceptibility to diseases.
"Mutations are the raw material of evolution, allowing species to adapt to changing conditions."
In summary, studying DNA mutations is essential for understanding the principles of genetics and evolution. Each type of mutation offers insights into genetic variability and the underlying mechanisms of biological functions. Through this examination, researchers can not only enhance our comprehension of hereditary processes but can also develop improved strategies for treating genetic conditions.
Genetic Variation and Evolution
Genetic variation is central to the understanding of evolution. It forms the foundation upon which natural selection and evolutionary processes act. Without variation among individuals, populations cannot evolve in response to changing environments. This concept is crucial for comprehending how species adapt, survival of the fittest, and the emergence of new species.
Genetic variations originate from mutations, gene flow, and sexual reproduction. Mutations can introduce new alleles into a population, while gene flow, or the movement of alleles between populations, can increase genetic diversity. Additionally, sexual reproduction creates new combinations of genes, enhancing variation within a population. The interplay of these factors leads to a rich tapestry of genetic diversity, which is essential for resilience and adaptability.
Researching genetic variation helps scientists understand and predict how populations respond to environmental pressures such as climate change or disease emergence. It can also lead to advancements in conservation strategies and agriculture. Understanding how genetic variation manifests in different populations provides insights into both human health and biodiversity.
"Genetic variation is not just a concept in a textbook; it is the thread that weaves the fabric of life and evolution."
Role of DNA in Evolution
DNA plays a role that is both fundamental and intricate in the process of evolution. It is the molecular basis for heredity, storing genetic information passed from one generation to the next. Each segment of DNA, or gene, carries instructions for traits that influence an organism's survival and reproduction.
Natural selection drives the process of evolution through variations in these traits, which can arise from mutations or genetic recombinations. For example, a mutation might create a trait that makes an organism better suited to its environment. If this trait offers a reproductive advantage, it may be passed on to offspring, resulting in a gradual shift in the population's genetic makeup over time.
Furthermore, DNA can reveal the evolutionary history of species through comparative genomics. By analyzing the DNA sequences of different organisms, scientists can construct evolutionary trees, elucidating common ancestors and the pathways of divergence.
Population Genetics
Population genetics is the study of genetic variation within populations and its evolutionary significance. It examines the frequency of alleles, genotypes, and phenotypes in a population, helping to understand how evolutionary forces like natural selection, genetic drift, and gene flow shape the genetic structure of populations.
Key concepts in population genetics include:
- Allele Frequency: The proportion of a particular allele among all allele copies in a population.
- Hardy-Weinberg Equilibrium: A principle that describes the genetic makeup of a population that is not evolving.
- Genetic Drift: Random changes in allele frequencies that can lead to significant changes in small populations.
Research in population genetics has applications in various fields, including ecology, conservation biology, and medicine. For example, it can inform conservation strategies by identifying genetically distinct populations that need protection. In medicine, understanding the genetics of populations can assist in tailoring public health interventions based on genetic susceptibilities to diseases.
DNA Technologies
DNA technologies are crucial for modern biology and medicine. They enable scientists to manipulate genetic material for various applications, from research to clinical settings. These technologies facilitate advancements in understanding genetic diseases, developing therapeutics, and exploring genetic diversity.
Understanding the techniques and methods of DNA technology is essential for appreciating its implications. This section will detail gene editing techniques and DNA sequencing methods, highlighting their unique characteristics and benefits.
Gene Editing Techniques
CRISPR-Cas9
CRISPR-Cas9 is a revolutionary gene-editing technology. It allows precise modifications in the DNA of living organisms. The key characteristic of CRISPR-Cas9 is its ability to target specific sequences of DNA. This precision makes it a popular choice for gene editing, not just in research but also in potential therapeutic settings.
A unique feature of CRISPR-Cas9 is its straightforward design and use. Researchers can customize CRISPR-Cas9 systems to edit genes by creating RNA sequences that guide the Cas9 enzyme to the desired DNA location. The advantages include efficiency and flexibility. However, there are concerns about off-target effects and long-term implications in genetic modifications.
TALENs
Transcription Activator-Like Effector Nucleases (TALENs) are another gene-editing tool. They function by creating double-strand breaks at specific DNA locations, similar to CRISPR. The primary advantage of TALENs is their high specificity. This makes them a favorable choice for certain complex genetic tasks.
A unique aspect of TALENs is the use of engineered proteins that bind to DNA. These proteins can be designed to target nearly any DNA sequence. While TALENs are effective, they can be more challenging to design and implement compared to CRISPR-Cas9. This complexity may limit their application in some areas of research.
Zinc Finger Nucleases
Zinc Finger Nucleases (ZFNs) are an older but still relevant gene-editing technique. Like TALENs and CRISPR, ZFNs introduce precise cuts in the DNA. The key characteristic of ZFNs is their modular design, which allows customization for specific DNA sequences.
A unique feature of ZFNs is their reliance on zinc finger proteins that recognize specific DNA triplets. Their main advantages include the ability to edit genomes with relatively high precision. Yet, ZFNs require a more intricate engineering process and can be less efficient than newer methods like CRISPR.
DNA Sequencing Methods
Accurate DNA sequencing is essential for various applications, including genomics and personalized medicine. Understanding these methods helps appreciate their role in advancing biological research.
Sanger Sequencing
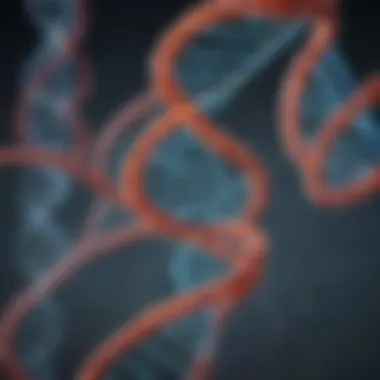
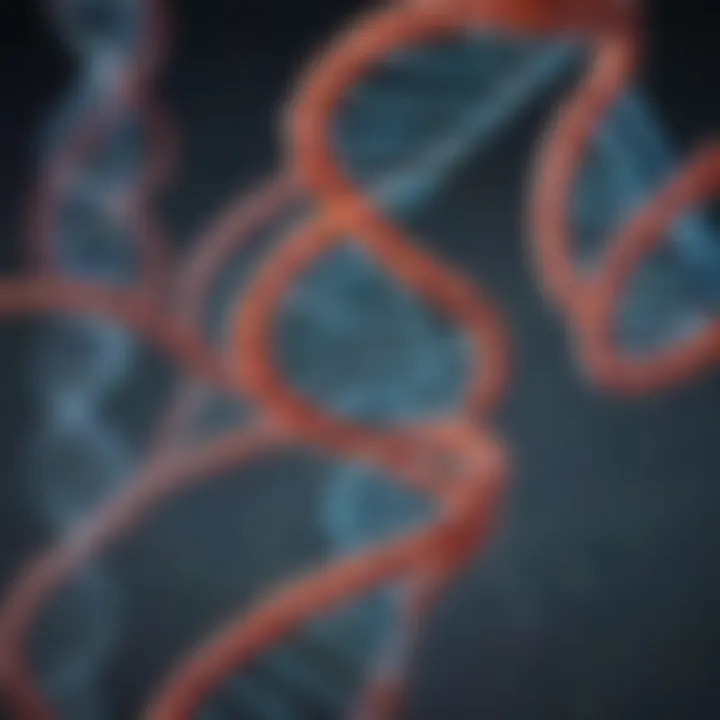
Sanger Sequencing is one of the first methods developed for determining the nucleotide sequence of DNA. Its key characteristic is the use of chain-terminating inhibitors, which allows for the identification of specific DNA fragments. This method remains beneficial in certain applications due to its high accuracy.
Sanger sequencing excels in applications requiring precise sequencing of shorter DNA strands. However, its limitations include longer processing times and higher costs compared to next-generation sequencing methods.
Next-Generation Sequencing
Next-Generation Sequencing (NGS) encompasses various modern sequencing technologies. It enables rapid and cost-effective sequencing of entire genomes. The key characteristic of NGS is its massively parallel processing capability, allowing for the sequencing of millions of fragments simultaneously.
A unique feature of NGS is its flexibility in applications, from whole-genome sequencing to targeted sequencing. The advantages include a significantly faster turnaround time and the ability to analyze larger datasets. However, data analysis can be complex and requires significant computational resources.
Applications of DNA Research
Applications of DNA research are pivotal in various fields, ranging from forensics to medicine. The advancements in understanding DNA's structure and function have opened new pathways for research and technology. This not only enhances scientific knowledge but also offers significant benefits to society.
Forensic Science
Forensic science utilizes DNA analysis in criminal investigations. The ability to match DNA samples from crime scenes with potential suspects has revolutionized the justice system. DNA profiling helps in identifying individuals with high precision, making it a crucial tool in solving crimes.
- Evidence Collection: DNA is collected from various biological samples, including blood, hair, and saliva, found at crime scenes. This material can provide conclusive evidence of a suspect's presence.
- Exoneration: DNA testing has helped in overturning wrongful convictions. This aspect underscores its power as a tool for justice.
- Database Utilization: National DNA databases hold profiles of known offenders. Such databases facilitate connections between crimes and suspects.
Using DNA in forensic analysis helps to bring clarity to ambiguities in criminal cases. It not only assists law enforcement but also empowers individuals within the legal system to make informed decisions based on scientific evidence.
Medical Genetics
Medical genetics represents another forefront of DNA research application, focusing on hereditary diseases and genetic disorders. Understanding the genetic basis of illnesses allows for targeted interventions and treatments.
- Genetic Testing: Patients can undergo genetic testing to identify predispositions to certain diseases. This knowledge aids in early diagnosis and careful monitoring.
- Personalized Medicine: Insights gained from DNA enable healthcare providers to tailor treatments. This customization enhances the effectiveness of medical therapies for individual patients.
- Gene Therapy: Innovative approaches, such as gene therapy, aim to rectify genetic defects at their source. Therapies can be designed using genetic information to treat or even cure various conditions.
"The future of medicine lies in understanding the genetic information provided by DNA."
This perspective emphasizes the profound implications medical genetics has on healthcare.
In summary, the applications of DNA research extend far beyond traditional boundaries. The influence on forensic science and medical genetics not only advances scientific knowledge but also contributes meaningfully to societal well-being. As research progresses, the potential of DNA will continue to expand, inviting new innovations and possibilities.
Ethical Considerations in DNA Research
Ethical considerations in DNA research have gained significant prominence as the field has evolved. As we unlock the mysteries of genetics and the potential applications of DNA technology, it is crucial to address the moral implications. These ethical questions surround areas such as genetic editing, privacy concerns, and the societal impacts of these advancements.
With the power techniques like CRISPR offer to alter genes, the ethical boundaries of genetic modification must be examined carefully. The ability to edit the human genome poses profound questions about what it means to be human. These questions include the potential for designer babies and the commodification of genetic traits. The challenge lies in balancing innovation with ethical responsibility.
Ethics of Genetic Editing
The introduction of genetic editing technologies, particularly CRISPR-Cas9, has opened new avenues in medical research, agriculture, and beyond. However, ethical dilemmas emerge about the limits of such modifications. Should we allow genetic changes that could potentially eradicate genetic diseases, or does this lead to unintended consequences?
There are arguments both for and against aggressive genetic editing.
- Proponents argue that these technologies can help eliminate serious genetic conditions, improving the quality of life for future generations.
- Opponents express concerns that editing the human germline may introduce unknown variables into the human gene pool, posing risks we cannot foresee.
In a world where genetic editing is feasible, establishing regulatory guidelines is crucial. Societies must discuss the ethical implications and determine appropriate boundaries guiding these technologies' use.
Privacy Concerns in Genetic Data
With advancements in DNA technologies, privacy concerns around genetic data are becoming increasingly significant. Individuals' genetic information contains sensitive information that can reveal not only personal health data but also familial relationships and ancestral backgrounds. This information can be misused or mishandled, leading to ethical breaches.
Several key points encompass privacy concerns, including:
- Data Security: Unauthorized access to genetic information can lead to discrimination by insurers or employers.
- Informed Consent: Individuals must be fully informed about how their genetic data will be used or shared with third parties. Clarity in consent processes is necessary to maintain trust.
- Anonymity: Maintaining the anonymity of individuals in genetic research is a pivotal concern. The intersection of genetic data and personal identity raises issues that must be carefully navigated.
Understanding these ethical concerns is essential for researchers, policymakers, and society as a whole to engage in informed debates about the future of DNA research.
Future Directions in DNA Research
The landscape of DNA research is rapidly evolving. This section examines the significance of future directions in DNA research, outlining advances that will shape our understanding and use of genetics. With biotechnology at the forefront, new techniques and methods are emerging. These developments promise to enhance our comprehension of DNA while also posing ethical considerations. It is essential to explore both the potentials and the challenges these innovations present.
Emerging Technologies
Recent years have seen remarkable advancements in emerging technologies relevant to DNA research. The most notable among these is the improvement in gene editing techniques. CRISPR-Cas9 remains a leading tool, allowing for precise alterations in the genetic sequence. This technology holds tremendous potential for correcting genetic disorders and customizing traits in organisms.
Other gene editing methods such as TALENs and Zinc Finger Nucleases also contribute to the toolkit available to scientists and researchers. Each of these tools functions on similar principles but vary in their mechanisms and effectiveness. The versatility of these technologies is driving innovations in synthetic biology and personalization of medical treatments.
In addition to gene editing, advancements in DNA sequencing methods are crucial. Technologies like Next-Generation Sequencing facilitate faster and more cost-effective sequencing compared to traditional methods like Sanger sequencing. This increase in efficiency allows researchers to analyze large volumes of genetic data, leading to discoveries that may have been previously unattainable.
Potential Impacts on Society
The impacts of advancements in DNA research extend far into society. As technologies improve, the possibilities in healthcare, agriculture, and environmental management grow. In the healthcare field, personalized medicine emerges as a leading application. Treatments tailored to an individualβs specific genetic makeup can lead to better outcomes. However, with this personalized approach comes the inevitable question of access and equity. How do we ensure that these advancements are accessible to all segments of the population?
Furthermore, ethical considerations regarding gene editing are paramount. Concerns about "designer babies" and the potential for genetic inequality arise in discussions about these technologies. Regulatory frameworks will need to catch up with science to prevent misuse.
"The future of DNA research is not merely about understanding our genetics; it is equally about defining the ethical boundaries within which these technologies must operate."
In agriculture, gene editing can improve crop resilience to climate change, which is a pressing global issue. However, the acceptance of genetically modified organisms (GMOs) among the public varies, leading to a social gap that researchers must navigate.
As DNA technologies continue to develop, so must our frameworks for ethical deliberation and societal responsibility. A significant challenge ahead will be to balance innovation with ethical restraint, ensuring that the benefits of DNA research are shared equitably across society.