Understanding the Fundamental Laws of Quantum Physics
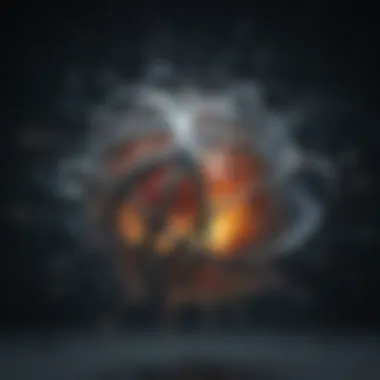
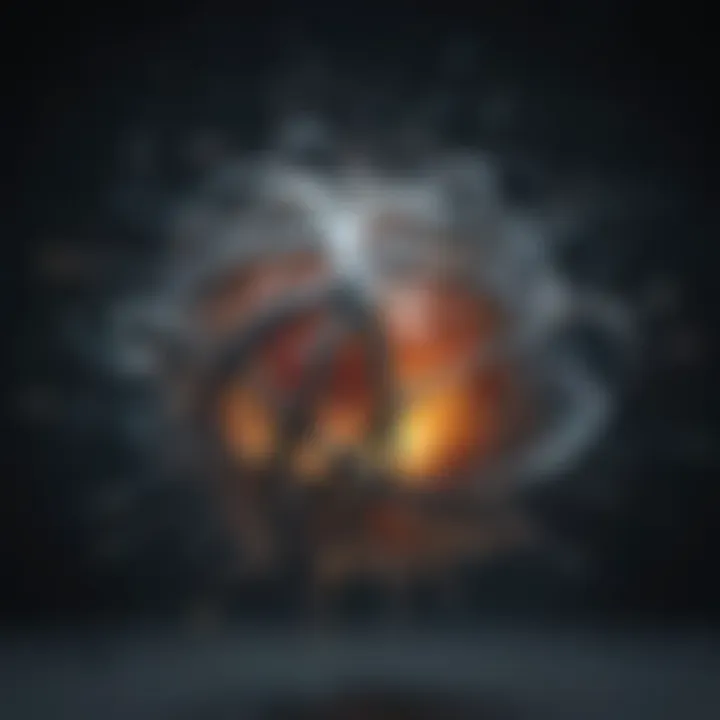
Intro
Quantum physics is a realm that challenges our traditional perceptions of nature. It shapes our understanding of the microscopic world, providing insights that contrast sharply with classical mechanics. As we traverse this intricate landscape, we encounter concepts that are both bewildering and profound. This article aims to unpack those intricacies, shedding light on the fundamental laws that govern quantum behavior.
Throughout the examination, we will delve into significant historical developments, pivotal experiments, and the underlying mathematical framework of quantum theory. We shall also identify its applications in modern technology and discuss the challenges it poses to classical intuitions. Understandably, this topic appeals to students, educators, and physics enthusiasts alike, who are eager to grasp the complexities of the quantum world.
Prologue to Quantum Physics
Quantum physics represents a fundamental shift in our understanding of the universe. It uncovers the behaviors and interactions of matter and energy at microscopic scales, where classical physics falls short. This field challenges conventional notions of reality, leading to profound implications not just in physics, but across all sciences.
Understanding quantum physics is essential for anyone investing in the future of technology and foundational sciences. The principles derived from this field provide insights that directly influence innovations in quantum computing, cryptography, and even sensors. Each concept reveals layers of complexity, often counterintuitive or even paradoxical to traditional frameworks.
In this article, we will explore the historical development of quantum physics, significant figures who shaped the field, and core principles that define it. This journey will equip readers with knowledge and perspective necessary to grasp the intricacies and applications of quantum mechanics.
Historical Background
The roots of quantum physics trace back to the early 20th century when scientists began to notice shortcomings in classical theories. Prior understandings struggled to explain phenomena like the black body radiation or the photoelectric effect. Planck's quantization of energy was a pivotal moment. His work laid the foundation for the entire field, suggesting that energy is not continuous, but rather composed of discrete packets called quanta.
Following Planck, Albert Einstein contributed significantly by explaining the photoelectric effect through the lens of quanta. His bold steps led to the theoretical framework that envisioned light not merely as a wave but also as a stream of particles, or photons. This duality became a cornerstone of quantum mechanics.
The early 20th century was marked by a flurry of breakthroughs from many notable scientists, including Niels Bohr, Werner Heisenberg, and Erwin Schrรถdinger. They all played crucial roles in building the theory of quantum mechanics, expanding its implications for atomic and subatomic particles. The historical context sets the stage for understanding current research and technological advances rooted in these foundational ideas.
Key Figures in Quantum Physics
Several individuals stand at the forefront of quantum physics, their names synonymous with transformative ideas.
- Max Planck: Recognized as the father of quantum theory, Planck introduced the concept of quantization of energy.
- Albert Einstein: Through his explanation of the photoelectric effect, he illustrated the dual nature of light.
- Niels Bohr: He complexified atomic models and introduced the principle of complementarity.
- Werner Heisenberg: Known for his uncertainty principle, it shaped the understanding that not all properties of particles can be simultaneously known.
- Erwin Schrรถdinger: Developed the wave equation that describes how the quantum state of a physical system changes over time.
These figures pushed the boundaries of scientific thought. Their collaborative efforts created a rich tapestry of ideas, paving the way for future explorations in theoretical and applied physics. Clearly, understanding their contributions is crucial for appreciating the entire landscape of quantum physics.
Core Principles of Quantum Mechanics
The core principles of quantum mechanics establish the framework that underpins our understanding of quantum phenomena. These principles are essential for grasping the behavior of particles at the subatomic level. They challenge traditional notions of physics, leading to revolutionary ideas that have shaped modern science. In this section, we will explore three key concepts: wave-particle duality, the quantum uncertainty principle, and quantum entanglement. Understanding these principles is crucial for both theoretical exploration and practical applications.
Wave-Particle Duality
Wave-particle duality is fundamental to quantum mechanics. This principle suggests that particles like electrons exhibit both wave-like and particle-like properties. At times, they behave as discrete particles, while at others, they demonstrate characteristics akin to waves. This duality is illustrated in the double-slit experiment, which shows how particles can create interference patterns, a property of waves, when not observed.
The implications of this principle are profound. It challenges the classical view of particles being solely one or the other. Scientists must consider the context in which a particle is measured to understand its behavior. This principle has led to advancements in various fields, revolutionizing technology in ways such as the development of semiconductors and quantum computing.
Quantum Uncertainty Principle
The quantum uncertainty principle, formulated by Werner Heisenberg, posits that certain pairs of properties, like position and momentum, cannot both be precisely measured at the same time. The more accurately one property is known, the less accurately the other can be determined. This principle is not merely a statement about measurement limitations; it reflects a fundamental aspect of nature.
This principle shakes the foundation of determinism that classical physics relied upon. It indicates a universe that is inherently probabilistic. In practical terms, this can affect predictions in fields such as quantum mechanics and even pave the way for advancements in quantum technologies that leverage uncertainty, such as quantum cryptography.
Quantum Entanglement
Quantum entanglement is another core principle that defies classical understanding. When particles become entangled, the state of one particle instantaneously influences the state of another, regardless of the distance separating them. This phenomenon suggests a unique kind of connection that cannot be explained by classical physics.
Entanglement has fascinating applications in quantum computing and quantum communication. It raises questions about the nature of information and connectivity in the universe. Experiments such as the Bell test have been conducted to investigate this phenomenon, confirming that entanglement exists despite being non-intuitive.
"Quantum entanglement challenges our understanding of reality, implying a level of interconnectedness that transcends classical limits."
In summary, the core principles of quantum mechanics shed light on the behavior of particles and their interactions. These principles not only revolutionize scientific understanding but also offer vast potential for future technological advancements. The ongoing research in this field continues to inspire curiosity and challenges conventional wisdom.
Mathematical Framework of Quantum Theory
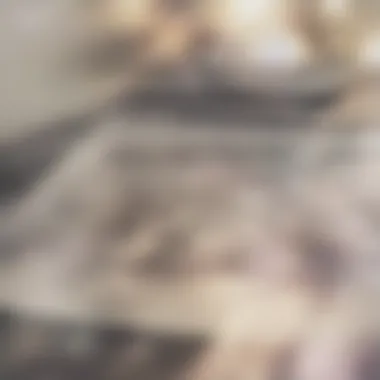
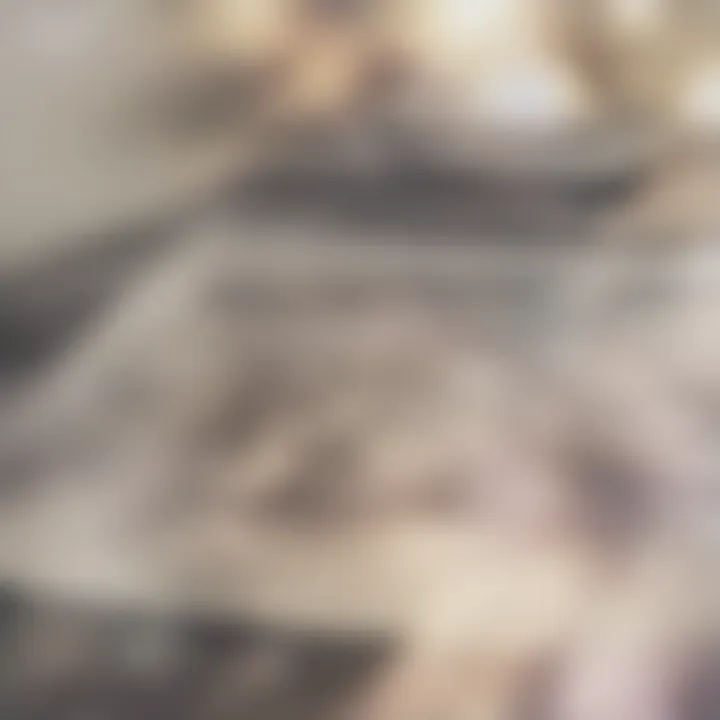
The mathematical framework of quantum theory is crucial for understanding the behavior of particles at the quantum level. This framework provides the necessary tools to describe quantum phenomena with precision. Understanding these concepts will enhance insights into quantum mechanics as well as its applications, making it key for students and professionals alike.
State Functions and Wave Functions
State functions are fundamental concepts in quantum physics. They offer a complete description of a quantum system. A state function, often represented by a vector in a complex vector space, encapsulates all possible information about a physical system. When we refer to wave functions, we are specifically talking about a particular type of state function applicable to quantum mechanics.
The wave function, denoted usually by the Greek letter psi (ฮจ), is essential for predicting the probabilities of finding a particle in various states. By squaring the absolute value of the wave function, one can derive the specific probability density associated with different positions in space. This probabilistic interpretation distinguishes quantum mechanics from classical physics, leading to notions of uncertainty and superposition.
Operators and Observables
In quantum mechanics, observablesโsuch as position, momentum, and energyโare represented by mathematical entities called operators. Operators act on state functions to extract measurable quantities.
When an operator is applied to the wave function, the result is related directly to the observable being measured. For example, the momentum operator yields information about the momentum of a particle when it operates on a wave function.
The correspondence between operators and observables illustrates the fundamental relationship between measurement and mathematical representation in quantum theory. This concept is both powerful and complex, forming the basis of how physical measurements are conducted in the quantum realm.
Schrodinger Equation
The Schrodinger Equation is often regarded as the cornerstone of quantum mechanics. It is a partial differential equation that describes how the quantum state of a physical system changes over time. The equation, expressed in its time-dependent form, is:
Here, (i) is the imaginary unit, (\hbar) is the reduced Planck's constant, (\Psi(x, t)) represents the wave function, and (\hatH) is the Hamiltonian operator responsible for total energy.
This equation highlights the dynamic nature of quantum states, emphasizing how energy influences behavior within systems. Understanding and solving the Schrodinger Equation is vital for predicting how quantum systems evolve, making it a critical component of the mathematical framework.
"The time-dependent Schrodinger Equation is the engine of quantum mechanics, providing insight into the dynamic behavior of quantum systems."
In summary, the mathematical framework of quantum theory lays the groundwork for comprehending the laws governing the quantum world. The interplay between state functions, observables, and the Schrodinger Equation works synergistically to form a comprehensive understanding of quantum mechanics.
Experimental Foundations of Quantum Physics
The experimental foundations of quantum physics serve as the bedrock upon which our current understanding of the universe builds. These experiments not only validate the theoretical frameworks but also challenge our classical intuitions. The significance lies in how they demonstrate the strange and often counterintuitive behaviors of particles at the quantum level. This section elaborates on some landmark experiments that have shaped quantum physics, illustrating their importance and implications.
Double-Slit Experiment
The double-slit experiment stands as a pivotal demonstration in quantum physics, revealing the dual wave-particle nature of light and matter. Initially conducted by Thomas Young in 1801, the experiment involved shining light through two closely spaced slits onto a screen. The resulting interference pattern suggested that light behaved as a wave.
With advancements in technology, similar experiments with electrons and other particles further solidified this finding. When particles are sent through the slits one at a time, they still produce an interference pattern, indicating that each particle interferes with itself as a wave. This challenges classical understandings of individuality in particles, suggesting a level of interconnectedness that eludes tangible reasoning.
Moreover, the introduction of a measurement mechanism alters the behavior of the particles. When observed, particles behave like discrete entities. This phenomenon underscores the concept of the observer effect, opening discussions about the implications of measurement in quantum mechanics.
Photoelectric Effect
The photoelectric effect presents another crucial aspect of quantum physics. First explained by Albert Einstein in 1905, this phenomenon observed that when light hits a metallic surface, it can eject electrons from that surface. The key insight was that light must exceed a certain frequency to release these electrons, regardless of its intensity. This finding linked the energy of photons to their frequency, establishing the concept of quantized energy levels.
Einstein's work on this effect earned him the Nobel Prize in Physics in 1921 and cemented the particle-like behavior of light. The photoelectric effect provides practical applications in various technologies, including photodetectors and solar panels.
This experiment is foundational for understanding light's dual nature and its interaction with matter. Without it, our understanding of electromagnetic radiation's behavior would remain incomplete.
Quantum Teleportation
Quantum teleportation is a more modern application, yet it has profound implications. This process does not involve the physical transfer of particles but rather relies on quantum entanglement. Two particles can be entangled such that the state of one immediately influences the state of another, regardless of the distance separating them.
In an experiment involving quantum teleportation, a particle's state can be transferred from one location to another without actually moving the particle itself. This relies on classical communication protocols and the entangled nature of the particles involved.
The implications of quantum teleportation are radical, suggesting potential advances in quantum computing, secure communication, and fundamental understandings of space and time. It challenges our concepts of locality and causality, making it a topic of ongoing research and debate.
"The experiments in quantum physics may lead to technologies we cannot yet conceive, reshaping how we understand information and reality itself."
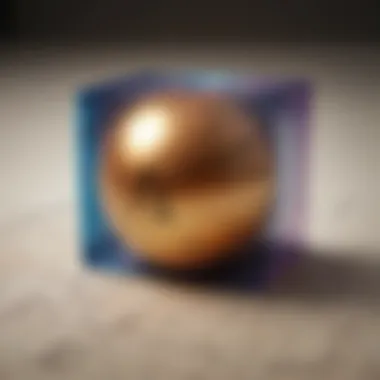
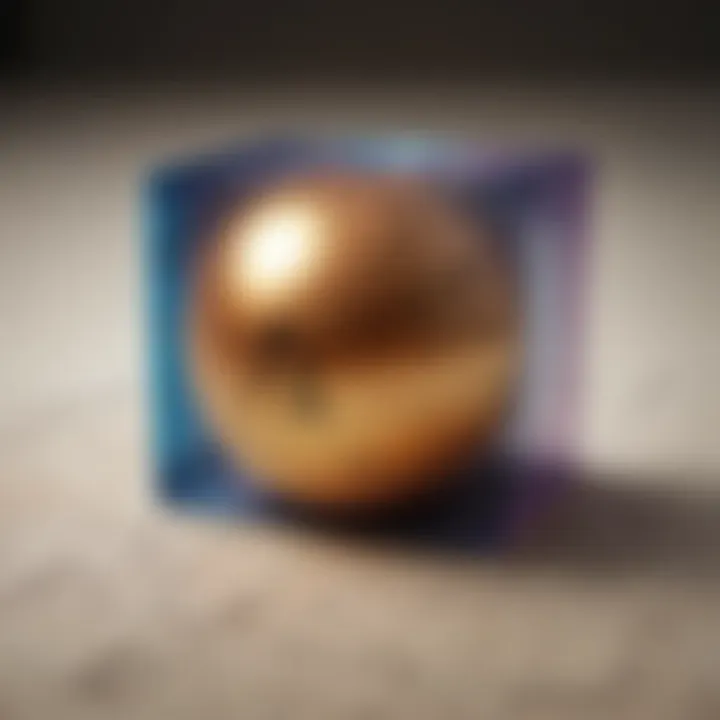
In summary, the experimental foundations of quantum physicsโillustrated through the double-slit experiment, photoelectric effect, and quantum teleportationโdemonstrate how rigorous testing and observation can unlock mysteries of quantum behavior. These experiments have paved the way for applications that drive modern technology and inspire future research.
Applications of Quantum Physics
The relevance of quantum physics extends well beyond theoretical discussions; it has profound implications in various fields of technology and science. The applications of quantum physics are transforming industries, shaping the future of computing, communication, and measurement, while also enhancing our understanding of complex phenomena. A clear grasp of these applications is essential for students, researchers, educators, and professionals, as they illustrate the practical benefits of quantum principles in real-world scenarios.
Quantum Computing
Quantum computing stands out as one of the most significant advancements derived from quantum mechanics. This technology exploits the peculiar properties of quantum bits, or qubits, which can represent both 0 and 1 simultaneously due to superposition. This capability allows quantum computers to perform calculations at an unprecedented speed. For certain types of problems, such as factoring large numbers or simulating molecular structures, quantum computers have the potential to outperform classical computers dramatically.
"Quantum computing is not just about speed; it changes the nature of what is computable."
Significant companies, including IBM and Google, are investing hugely in research and development for quantum processors. The implications for fields such as cryptography, optimization, and drug discovery are immense, showcasing how quantum computing could solve problems deemed intractable with current technology. However, challenges remain, particularly in error correction and scaling up qubit systems, which researchers are addressing through ongoing work in the field.
Quantum Cryptography
Quantum cryptography offers a revolutionary approach to secure communication. Utilizing principles like quantum entanglement and the uncertainty principle, this application promises to create secure channels for transmitting information. Quantum Key Distribution (QKD) is a prime example, allowing two parties to generate a shared, random secret key that is secure from any eavesdroppers.
One notable protocol is the BB84 algorithm, which uses the properties of light and measurements to ensure that any interception of the key will be detectable by the communicating parties. Organizations like the Quantum Safe Cryptography Consortium are exploring how quantum cryptography can safeguard sensitive information against future threats posed by quantum computers. It opens pathways to a level of security that classical systems cannot achieve, transforming how we protect data integrity in our interconnected world.
Quantum Sensors
Quantum sensors leverage quantum mechanics to achieve unprecedented sensitivity in measurement. These devices can measure physical quantities such as time, acceleration, and magnetic fields with remarkable precision. For instance, atomic clocks, which rely on the vibrations of atoms, are already a crucial technology in GPS systems and telecommunications.
Another example includes quantum magnetometers, which are useful in medical imaging, such as magnetoencephalography (MEG), a non-invasive technique to measure the magnetic fields produced by neural activity in the brain. The potential applications span across various fields, including geology, national defense, and environmental monitoring.
As researchers continue to harness quantum technologies, the evolution of quantum sensors is anticipated to open new frontiers in scientific research and practical applications, pushing the boundaries of what we can measure and understand.
Philosophical Implications of Quantum Physics
Quantum physics extends beyond mere experimental science; it raises profound philosophical questions that challenge our understanding of the universe. This section examines the implications of quantum mechanics, particularly its influence on concepts such as reality, determinism, and the role of the observer. Such discussions are crucial in a time when traditional views of physics are being re-evaluated in light of new scientific data and interpretations.
Determinism vs. Indeterminism
At the heart of quantum theory lies a significant debate between determinism and indeterminism. Classical physics, particularly Newtonian mechanics, relies on deterministic principles. According to determinism, given complete knowledge of a system at one point in time, one could predict its future state with absolute certainty. In contrast, quantum mechanics introduces an element of indeterminism. The principle of quantum uncertainty dictates that certain pairs of physical properties, known as complementary variables, cannot be simultaneously known to arbitrary precision. A common example is the position and momentum of a particle.
This inherent uncertainty suggests that, at a fundamental level, the universe does not adhere strictly to deterministic laws.
- Key points in the debate:
- Historical reliance on determinism in classical physics.
- Quantum mechanics challenges this view.
- Implications for predictability and causality.
The implications of this shift are profound. They force us to reconsider not only how we understand motion and change but also what it means to know something about the universe. If events at the quantum level are inherently unpredictable, what does this mean for our concept of free will?
The Observer Effect
The observer effect is another critical concept within the philosophical implications of quantum physics. This principle states that the mere act of observing a quantum system can alter its state. It is not merely a technical challenge; it poses significant questions about the nature of reality itself.
In essence, when an observer measures a quantum particle, it forces the particle to 'choose' a definite state. Prior to measurement, the particle exists in a superposition of states. Once observed, the particle collapses to one of the possible outcomes.
- Considerations of the observer effect:
- Reality is not independent of observation.
- Raises questions about the role of the observer in creating reality.
- It insinuates a fundamental connection between consciousness and the physical world.
This idea prompts philosophical inquiries into the nature of reality and perception. Does reality exist independently of our observation? Or does it need an observer to manifest? These questions tie into broader themes regarding consciousness and existence.
"The act of observing can fundamentally change the nature of what is being observed."
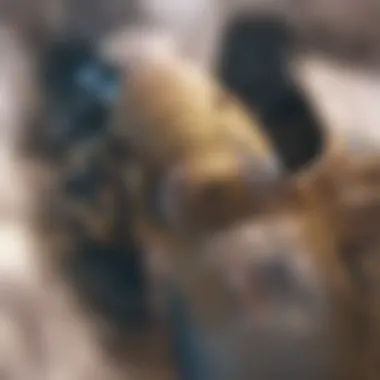
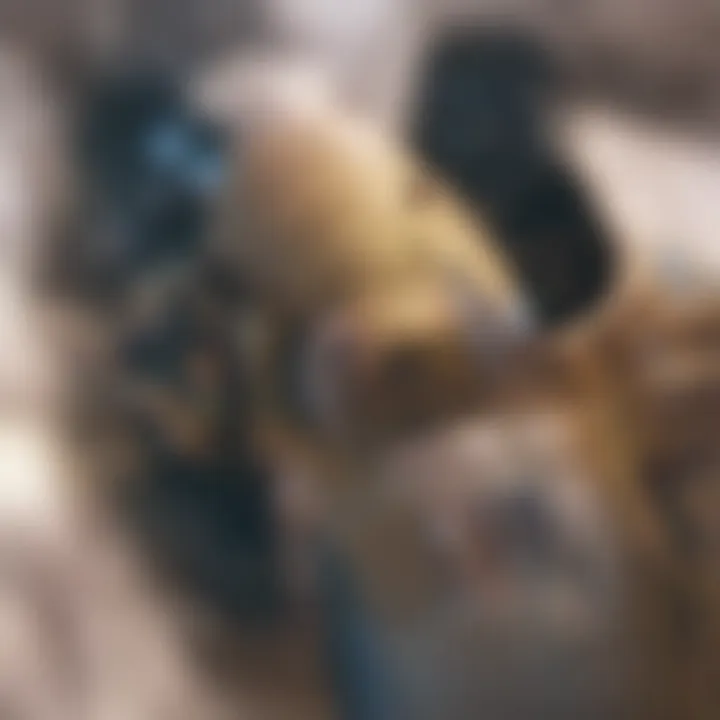
These philosophical considerations underline the importance of examining quantum mechanics not just as a scientific theory but as a framework that reshapes our understanding of existence itself. This dimension emphasizes that quantum physics is not just about particles and waves; it poses questions that merge science with philosophy, thereby enriching both fields.
Challenges in Quantum Physics
The realm of quantum physics is not without its challenges. These difficulties often appear at the intersection of theoretical interpretation and experimental practice. Understanding these challenges is essential as it addresses the gaps that still exist in our comprehension of the quantum world.
Interpretations of Quantum Mechanics
The interpretations of quantum mechanics serve as fundamental discussions among physicists. Since the advent of quantum theory, numerous interpretations have emerged to explain the mathematical formalism. Key interpretations include the Copenhagen Interpretation, many-worlds interpretation, and de Broglie-Bohm theory. Each of these perspectives presents unique philosophical implications about reality, measurement, and determinism.
- Copenhagen Interpretation: This is perhaps the most famous interpretation, positing that physical systems do not have definite properties until measured. This creates a dualistic nature between the wave function and reality.
- Many-Worlds Interpretation: This approach suggests that all possible outcomes of quantum events actually occur in branching, parallel universes.
- De Broglie-Bohm Theory: This is a deterministic interpretation that introduces hidden variables to restore classical determinism.
These interpretations highlight the ongoing debate in quantum mechanics and challenge the fundamental notions of causality and reality.
Experimental Limitations
Experimental limitations are another significant challenge in quantum physics. Despite theoretical advancements, practical experimentation often encounters obstacles that can skew data or limit conclusions. Key aspects include:
- Measurement Precision: Quantum systems are frail; slight external influences can affect outcomes. The challenge of achieving precise measurements is a persistent hurdle.
- Scalability of Experiments: Many quantum experiments are conducted on a small scale, and scaling these results to larger systems presents complications.
- Technological Constraints: As technology progresses, some experimental setups become obsolete. New technologies can provide better insights, but existing tools may limit understanding.
The challenge lies not only in collecting data but also in interpreting it accurately while considering these limitations. Addressing these challenges is critical for advancing the field.
Future Directions in Quantum Research
The landscape of quantum research is continuously evolving. Exploring future directions is essential because this field holds immense potential to redefine technology and our understanding of fundamental laws. There are specific elements worth discussing in this context, particularly advancements in quantum technologies and the significance of cross-disciplinary research.
Advancements in Quantum Technologies
Quantum technologies are making strides that could revolutionize various sectors. Innovations in quantum computing, for instance, promise to solve complex problems too intricate for classical computers. Companies like Google and IBM are actively developing quantum processors to harness the principles of quantum mechanics. These efforts could enable significant breakthroughs in fields such as cryptography and material science.
Moreover, quantum communication is gaining traction. Methods to achieve ultra-secure communication channels through quantum key distribution illustrate how quantum principles can enhance cybersecurity. The importance of these advancements lies in their capacity to increase computational efficiency and enhance data security, crucial elements in our data-driven society.
Cross-Disciplinary Research
Cross-disciplinary research is crucial for the advancement of quantum physics. Collaborations between physicists, computer scientists, and engineers foster innovation. For example, integrating insights from computer science can enhance algorithms used in quantum computing. Likewise, insights from engineering can lead to better physical implementations of quantum technologies.
Considering the complex nature of quantum mechanics, blending knowledge from different domains encourages new approaches to tackling current challenges. It also broadens the applicability of quantum principles across industries such as healthcare, finance, and telecommunications. Thus, fostering a cross-disciplinary environment enhances the research's relevance and potential impact.
"Quantum research is not just a niche field anymore; it is increasingly becoming a cornerstone for innovation across various disciplines."
Exploring these future pathways is essential for students, researchers, educators, and professionals who wish to engage with the ever-expanding horizons of quantum science.
Closure
The conclusion of this article encapsulates the expansive universe of quantum physics, emphasizing its significance in both theoretical and applied contexts. This field, with its intricate laws and principles, has profound implications that extend beyond the realm of academia. Understanding these complexities is essential, not just for researchers and theorists, but also for the next generation of scientists and technologists.
Examining the laws of quantum physics leads to a greater appreciation of the natural world. Quantum mechanics informs a myriad of technologies, from semiconductors to advanced medical imaging equipment, fundamentally shaping modern life. The interplay of theory and application exemplified in quantum mechanics serves as a crucial case study in the evolution of scientific understanding.
Summary of Key Points
The discussion throughout this article has highlighted several core elements of quantum physics:
- Historical development: tracing back to the early 20th century, key milestones and figures that shaped the discipline.
- Core principles: such as wave-particle duality and the uncertainty principle, which challenge classical understandings.
- Mathematical frameworks: the essential role of state functions and the Schrรถdinger equation in modeling quantum systems.
- Experiments: foundational experiments including the double-slit experiment and the photoelectric effect solidifying the principles of quantum mechanics.
- Applications: real-world utilizations of quantum physics in computing, cryptography, and sensor technology, demonstrating its practicality.
- Philosophical implications: addressing how quantum physics confronts notions of determinism.
- Future directions: exploring advancements that may redefine technology and scientific inquiry.
Impact on Future Generations
The implications of quantum physics are poised to inspire and inform future generations. As our understanding deepens, the next wave of scientists will have the responsibility of continuing this exploration. The ethical considerations surrounding quantum technologies, such as quantum cryptography, present new challenges to navigate.
Moreover, the integration of quantum physics into education will equip students with the tools necessary to tackle complex scientific problems. Interdisciplinary research may lead to innovations whose potential we have yet to fully realize. Quantum physics, therefore, is not merely a branch of science but a lens through which to view and interpret the world. Its evolving nature ensures that its contributions will resonate for decades to come.
"The future of quantum physics holds both challenges and immense potential, urging us to remain inquisitive and reflective about its profound mysteries."
Overall, comprehension of the laws of quantum physics is vital in comprehending the fabric of modern science and technology, shaping not only the field itself but also the course of human progress.